To Issue 170
Citation: Jameson H, Farrow D, “Blisters, Bonds and Biologics: How to Achieve Stability of Dry Powder Biologics”. ONdrugDelivery, Issue 170 (Apr 2025), pp 24–28.
Heather Jameson and Dave Farrow cover the challenging formulation science required to develop a viable dry powder aerosol and examine the inhaler device requirements necessary to protect the formulation throughout its shelf life while also ensuring consistent, high-performance delivery.
“AS TECHNOLOGY ADVANCES AND UNDERSTANDING OF DISEASE MECHANISMS DEEPENS, DRY POWDER BIOLOGICAL AEROSOLS ARE POISED TO PLAY AN INCREASINGLY KEY ROLE IN MEDICINE, OFFERING TARGETED THERAPIES WITH IMPROVED EFFICACY AND REDUCED SIDE EFFECTS.”
Dry powder biological aerosols hold immense potential to transform the treatment of respiratory and systemic diseases.1,2 This rapidly evolving field is driven by unmet medical needs, promising clinical results and significant investment in research and development. As technology advances and understanding of disease mechanisms deepens, dry powder biological aerosols are poised to play an increasingly key role in medicine, offering targeted therapies with improved efficacy and reduced side effects.
However, solid-state biological aerosols are fiendishly difficult to develop into drug products, requiring a strong synergistic programme involving formulation science and delivery device technologies. Additionally, biologic formulations are highly sensitive to environmental conditions, so choosing an inhaler device with the right primary packaging is essential.
Various biological modalities are being investigated and developed for aerosol delivery, each with unique properties and therapeutic applications (Table 1). These modalities are often selected for dry powder delivery due to their suitability for this format or for the advantages that a dry powder format might bring. Dry powder inhalers offer advantages such as portability, ease of use and improved stability compared with liquid formulations.
Modality | Properties | Applications |
Peptides | Short chains of amino acids, target specific receptors, modulate cellular processes | Asthma, pulmonary hypertension, cystic fibrosis |
Proteins | Larger and more complex than peptides, crucial roles in biological processes | Cystic fibrosis, respiratory infections, tissue regeneration |
Antibodies | Highly specific molecules, target and neutralise pathogens, modulate immune responses | Respiratory infections, including covid-19 |
mRNA | Genetic material, encodes proteins, used in vaccines and protein replacement therapies | Cystic fibrosis, genetic disorders, vaccines |
Table 1: Types of biological modalities.
FORMULATING BIOLOGICAL DRY POWDERS FOR INHALATION
Solid Versus Liquid State Most biological modalities exist in a native liquid state, so converting them to a powder requires a drying step. While this can be complex, the possible advantages of dry powder aerosol products can be significant compared with the liquid versions.
There are cases when a liquid aerosol product works perfectly well and is a superior format. However, there are many cases, principally for high doses or for stability reasons, where a dry powder is needed to form a viable product.
Dry Powders for Inhalation
Producing dry powders for inhalation can significantly affect protein aggregation behaviour. The impact of the drying process alters the biologic molecule’s energy state, resulting in increased aggregation, fibrillisation, degradation, oxidation and other processes that can compromise stability, quality and bioavailability.
To be viable for inhalation, particles with a mass median aerodynamic diameter (MMAD) of typically less than 5.0 μm must be produced. Currently, there are two main methods that offer effective drying, particle size control and proven cGMP scalability – lyophilisation and spray drying – although there are many innovative technologies under development.
Lyophilisation, or freeze drying, is a widely used technique for stabilising biological modalities, particularly proteins and peptides. This process involves removing water from the formulation under low temperature and pressure. The powder first forms as a solid cake, which must then be ground down (a process called comminution) to achieve a powder with the required size and distribution. This process can involve high energies that can destroy biological modalities.
Spray drying, on the other hand, involves spraying a liquid formulation into a hot chamber, where the water or other solvent media rapidly evaporates, leaving behind a dry powder. Spray drying can be a faster and more cost-effective alternative to lyophilisation for some applications but can also be more complex in some respects.
For aerosols, spray drying has some distinct advantages. These include:
- Control over particle size
- Single-step process (no comminution step)
- Uniform spherical morphology
- Potential to engineer particles to have a force control agent surface coating to improve aerosolisation
- Readily form amorphous matrices (which is necessary for the complex biological structures).
Water Pinning
Unlike small-molecule drugs, biologics often have complex secondary, tertiary and quaternary structures that are intrinsic to their therapeutic activity (Figure 1). Biologic modalities usually exist in the liquid state and water plays a crucial role in keeping these complex structures intact.
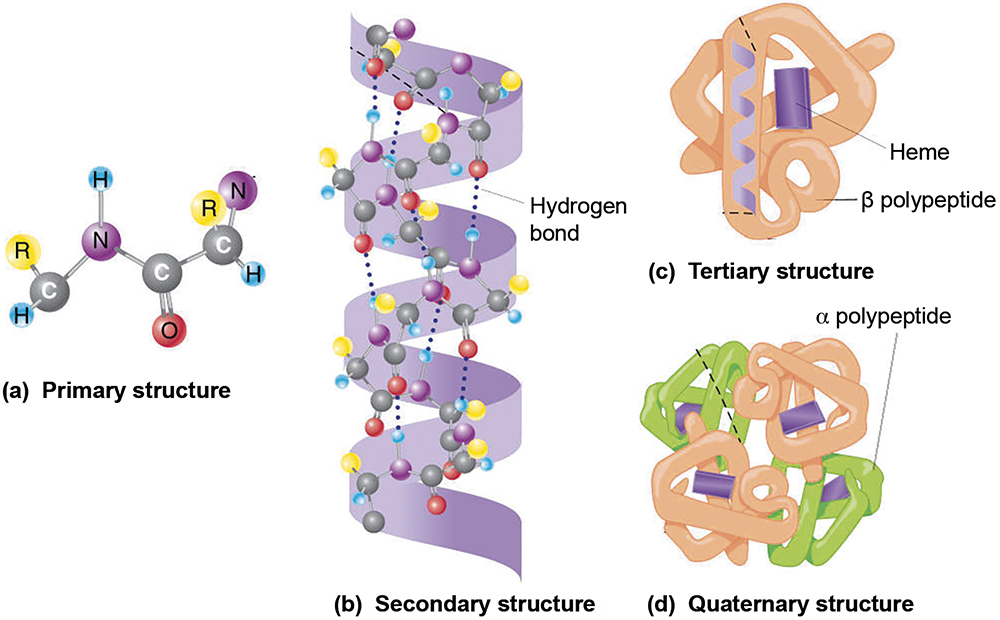
Figure 1: Biologics often have complex secondary, tertiary and quaternary structures that are intrinsic to their therapeutic activity.
Water binds to the surface of the biomolecule at certain specific points. Imagine a dressmaker pinning folded cloth to a dummy to make the dress – in this analogy the water acts as the pins (Figure 2). The cloth material is composed of the hydrogen bonding locking the fibres together, but it only takes a functional form when the water pins hold the shape. Therefore, any drying step that removes this water risks the loss of activity of the biomolecule. Luckily, there are mechanisms that allow the structures to be preserved in the drying process as the water is removed.
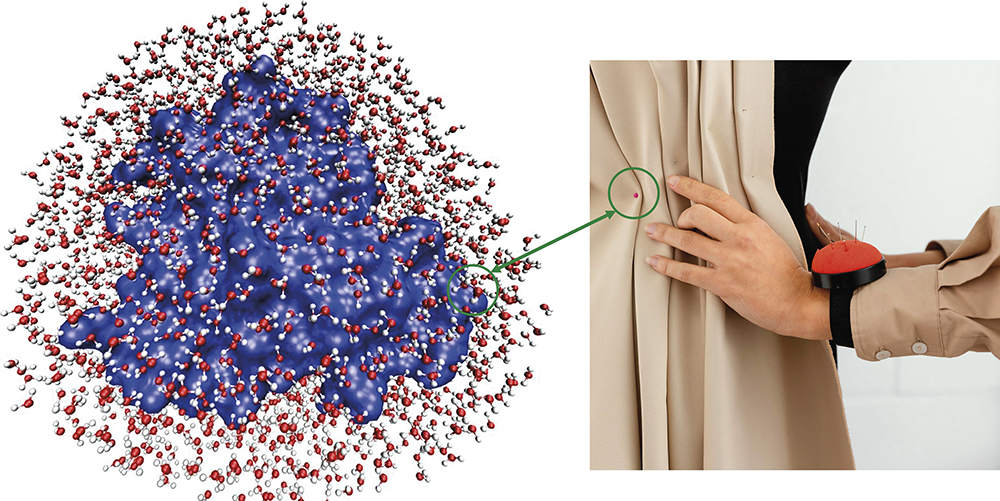
Figure 2: Water molecules play a crucial role in maintaining the complex biologic structures, like a dressmaker using pins to hold the fabric in place.3
Solid-State Stabilisation
Before drying, biologic molecules are dissolved in an aqueous solution containing stabilising excipients. These excipients, often sugars such as trehalose or sucrose, help protect the protein’s structure during dehydration and act as stabilising agents in the final powder.
As water is removed during the drying process, the biologic molecules must be stabilised within an amorphous matrix.4 Two key stabilisation mechanisms occur (Figure 3):
- Mechanical Entrapment (Vitrification Theory): The solid, glass-like matrix is prepared, physically immobilising the biologic and preventing molecular motion. This protects the biomolecule from degradation by locking it in place in a rigid, amorphous structure.
- Water Entrapment (Hydrogen Bonding Theory): A small amount of bound water remains attached to the biomolecule, preserving its structure. Rather than forming hydrogen bonds directly with the excipient, the protein is coupled to the amorphous matrix through water molecules entrapped at the interface.
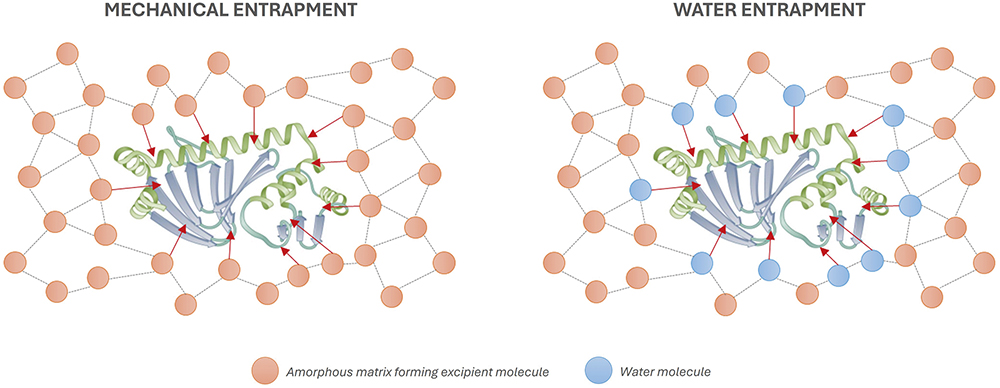
Figure 3: Two stabilisation mechanisms – mechanical entrapment and water entrapment.
Although they are physically different mechanisms, both achieve the goal of supporting the biologic structure within an amorphous matrix via hydrogen bonding. Both mechanisms can occur simultaneously, with the balance between the mechanisms being influenced by the choice of excipients and the spray-drying conditions, such as temperature, feed rate and concentration.
Thermodynamic Stability
The amorphous matrix, categorised by a disordered molecular arrangement, forms around the surface of the modality. It needs to be amorphous to form a flexible format, ensuring conservation of the higher-order structure. It is critical that the matrices do not crystallise, as this would crush and distort the biomolecule, leading to structural degradation and significant loss of therapeutic activity.
However, an amorphous matrix is not thermodynamically stable. They desire to be crystalline. The Gibbs free energy of an amorphous state is higher than that of a crystalline state, due to the higher entropy and enthalpy of the amorphous state, making crystallisation a thermodynamically favoured process (Figure 4).
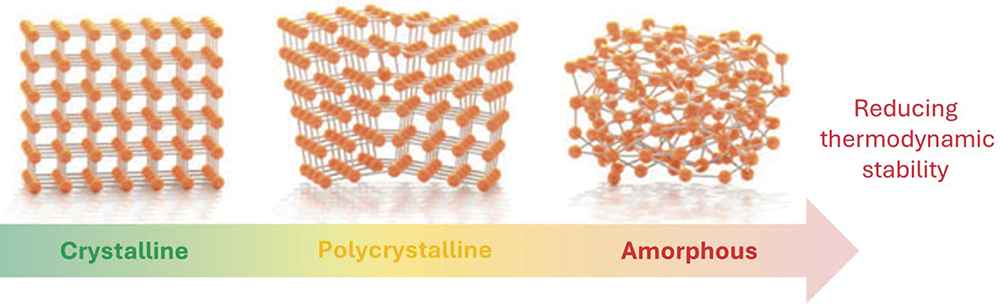
Figure 4: The amorphous state has higher Gibbs free energy than the crystalline state due to its greater entropy and enthalpy, making crystallisation thermodynamically favoured.
Keeping an amorphous matrix stable and preventing crystallisation is a key challenge for formulators. Amorphous matrices are highly hygroscopic, meaning that they readily absorb moisture. For crystallisation to occur, two factors are needed:
- Heat, to break hydrogen bonds
- A solvent, such as water, to help molecules rearrange into a crystalline structure.
Water, with its strong hydrogen bonding properties, can lower the energy needed for crystallisation and disrupt the amorphous structure on its own, which is a major concern for inhalable dry powders. The particles are carefully designed for optimal lung delivery, so they must be protected from moisture at all costs. Therefore, selecting the correct inhaler device and associated primary packaging closure system is crucial to achieving the promise of inhaled biologic therapies.
PRIMARY PACKAGING SYSTEMS AND THEIR SUITABILITY FOR BIOLOGIC AEROSOLS
Inhaler devices can be categorised into three groups based on the primary packaging of the formulation: reservoir, capsule and blister devices. Due to their inferior inherent moisture ingress protection, reservoir devices will not be discussed here.
Capsules Versus Blisters
Capsule devices are generally unit-dose devices, such as Berry Global’s RS01, where the user loads a capsule into the device for each dose. Gelatine was the industry-standard material for capsules, but it is now being phased out in favour of hydroxypropyl methylcellulose (HPMC). Capsules have minimal inherent moisture ingress protection, so the capsule is stored in a blister until needed. This has several disadvantages compared with storing the formulation directly in a blister:
- Additional cost of capsule plus blister
- Additional user steps of removing the capsule from the blister and inserting into the device
- Increased time between piercing the blister and inhalation of the formulation.
For biologic formulations, which are extremely sensitive to moisture ingress, several minutes of exposure to atmospheric humidity may be too long, particularly in humid climates. Furthermore, the conditions within the blister must be carefully controlled. If the environment inside the blister is too dry, the capsule shell becomes brittle and fragile, making it difficult to pierce as the capsule may shatter instead of opening cleanly.
Blister Sealing Considerations
A well-designed blister device is one of the best solutions for delivering biologic aerosols to the lung, due to the superior moisture ingress protection of blisters, which protect the formulation right up until the moment of actuation. Moreover, a breath-actuated mechanism can be incorporated, which ensures that the blister is only breached after the patient starts inhaling.
There are two primary mechanisms for “opening” the foil of the blister: pealing or piercing. Devices such as GSK’s Diskus and Ellipta incorporate a mechanical system that peels open the foil to expose the dose at the right moment before inhalation. Peeling back the foil has the advantage that the foil is moved out of the way, meaning that it cannot impede the aerosolisation of the powder.
However, to ensure that the foil does not rupture when peeled back, the strength of the seal must be intentionally limited, which limits the moisture protection. Therefore, to achieve the best moisture protection, the foil must be pierced. With considered piercer design, the pierced foil flaps can be folded out of the way of the airflow.
Blister Size and Shape
If blisters are to be pierced, then there must be space left inside the blister for the piercers to protrude into the blister volume. This means that the blisters cannot be brim-filled. Although this requires the volume of the blisters to be larger, allowing for head space in the blister makes the filling process easier and the sealing more robust, due to reduced risk of powder spilling onto the sealing surface.
Inhaled biologics require higher doses than traditional inhaled therapies for asthma and COPD, which will also necessitate larger blister cavities to accommodate more powder (Figure 5). Furthermore, engineered powders with small particle size distributions are cohesive (Geldart Class C) and pack inefficiently, leading to low densities.
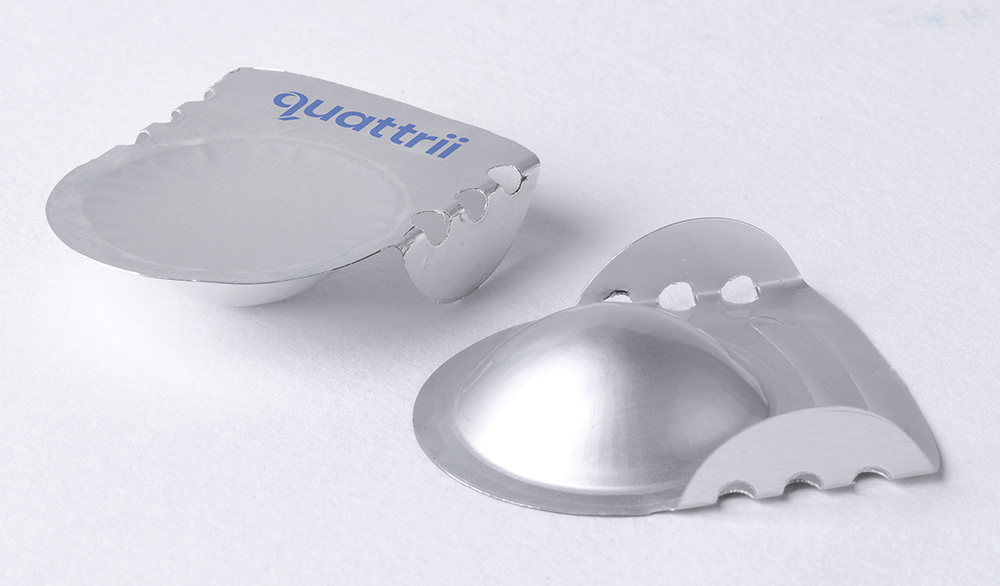
Figure 5: An example of a large-volume blister (1,414 μL).
“THE OPTIMUM BLISTER CAVITY SHAPE MAXIMISES VOLUME WHILE MINIMISING SURFACE AREA, THE AIM BEING TO MINIMISE
THE AREA ONTO WHICH POWDER CAN BE DEPOSITED AND RETAINED – WHICH IS ESPECIALLY IMPORTANT FOR
CARRIER-FREE FORMULATIONS.”
The optimum blister cavity shape maximises volume while minimising surface area, the aim being to minimise the area onto which powder can be deposited and retained – which is especially important for carrier-free formulations. Maximising blister depth is also important to allow height for piercers to protrude into the blister volume. Sharp piercers are required to ensure reliable clean piercing without rupturing the foil, but generally require greater height than more blunt shapes.
On the other hand, over-stretching the coldform will lead to loss of moisture protection, due to the aluminium layer rupturing. Typically, for standard three-ply coldform, the stretch should be limited to less than 32%. A paraboloid is the most efficient blister shape, achieving the maximum volume and depth for a given diameter, while staying below the critical 32% stretch.
Blister shape also influences airflow patterns, affecting how powder is delivered to the patient. Efficient airflow is critical for aerosolisation and overcoming interparticle forces. Highly cohesive engineered particles require significant aerosolisation power to achieve effective deagglomeration. Both high-velocity airflows and high-momentum collisions of particles enhance deagglomeration.
Through considered, synergistic design of the piercers, airflow inlets and blister shape, an optimised cyclonic flow pattern can be established within the blister to achieve efficient aerosolisation and deagglomeration of solid-state biologic engineered powders.
CONCLUSION
The possibility and opportunity to develop engineered biological dry powders is significant, but so are the formulation challenges. The formulations themselves are thermodynamically unstable and prone to degradation or particle agglomeration over time. Making sure that the carefully and precisely engineered powder particles remain stable over the lifespan of the product is critical.
Engineered dry powder biologic formulations are particularly sensitive to moisture ingress. A well-designed large-volume blister-based inhaler device is the optimal solution for delivering biologic aerosols to the lung, due to the superior moisture ingress protection of blisters and the dose requirements. However, not all blister designs are created equal, and considered design is needed to achieve optimum stability and aerosolisation performance.
REFERENCES
- Sécher T, Heuzé-Vourc’h N, “Pulmonary Delivery of Antibody for the Treatment of Respiratory Diseases” in “Respiratory Delivery of Antibody for the Treatment of Respiratory Diseases” (Lam J, Kwok PCL eds). AAPSINSTR Vol 8, Springer, 2023.
- Ecenarro Probst S, Buttini F, “Inhalable Therapeutic Biologics, a Paradigm Shift for Non-Invasive Efficient Medical Treatments”. ONdrugDelivery, Issue 92 (Nov 2018), pp 40-44.
- Frauenfelder H et al, “A unified model of protein dynamics”. Proc Natl Acad Sci USA, 2009, Vol 106(13), pp 5129–5134.
- Shepard KB et al, “Dry Powder Formulation of Monoclonal Antibodies for Pulmonary Delivery” in “Respiratory Delivery of Antibody for the Treatment of Respiratory Diseases” (Lam J, Kwok PCL eds). AAPSINSTR Vol 8, Springer, 2023.