To Issue 160
Citation: Rothhaar U, Haines D, Bicker M, Schmidt T, “Packaging Material Identification to Ensure Selection of Best-Matching Components for Parenteral Drugs”. ONdrugDelivery, Issue 160 (May 2024), pp 81–88.
Uwe Rothhaar, Daniel Haines, Matthias Bicker and Thomas Schmidt discuss the analytical techniques used to identify the material components and composition of primary containers for injectable drug products.
“A key responsibility of pharmaceutical companies is to ensure that the actual quality of packaging components supplied by component manufacturers meets compendial requirements and matches the agreed upon specifications.”
Drug container closure and delivery systems are constructed from components made from various material classes, including glass, rubber and plastic, in combination with several other aspects, such as coatings, treatments and process refinements, that provide special properties and functional performances. A key responsibility of pharmaceutical companies is to ensure that the actual quality of packaging components supplied by component manufacturers meets compendial requirements and matches the agreed upon specifications. Especially critical are the materials and surfaces in direct contact with the drug product, which can have a large influence on its stability and shelf life. Therefore, the characterisation of packaging components with respect to the compositions of the used materials, coatings and/or refinement processes is an important factor in ensuring the quality and performance of the complete pharmaceutical packaging system.
SCHOTT Pharma Services provides packaging material identification studies (PMIS) that support the pharmaceutical industry in confirming material specifications from component vendors, via either lot-to-lot or yearly supplier verification, or in elucidating the component material and surface compositions of already marketed products. In addition, these studies can support the troubleshooting of drug container compatibility issues such as protein adsorption or aggregation, pH shift, glass delamination, leaching of elemental impurities and other drug stability issues that may arise from the use of different primary packaging components and materials.
A typical set of aspects considered in a PMIS is shown in Figure 1. In these studies, a set of different analytical techniques is used to gain insights into the different base materials used and to reveal surface functionalisation by, for example, siliconisation, coatings, ion-exchange or de-alkalisation. The results are then compared with in-house databases of published compositions and reference materials. Combining this set-up with extensive expertise in drug packaging design, development and characterisation enables the identification of properties specific to the chosen containment solution design.
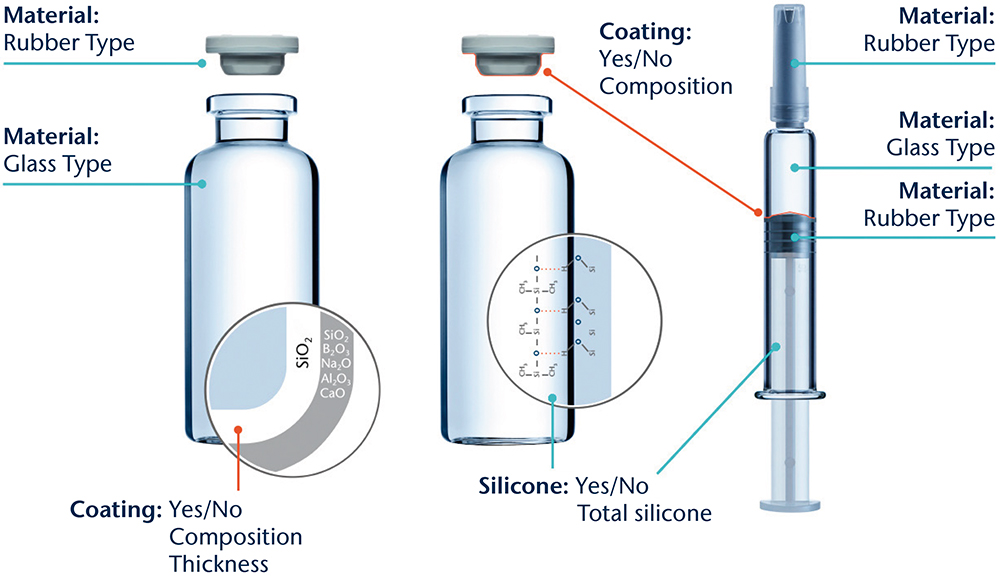
Figure 1: Illustration of the different aspects to be considered for a PMIS.
GLASS CONTAINERS
Glass is by far the most common container material used where there is direct contact with parenteral drug products. Up until September 30, 2023, the US Pharmacopeia (USP) <660> and European Pharmacopoeia (Ph Eur) 3.2.1. defined pharmaceutical glass as either a borosilicate (neutral) or a soda-lime-silica type. Based on the results of tests described in those monographs, the glass containers are classified as Type I, made of borosilicate glass; Type II, made of soda-lime-silicate with a surface treatment (de-alkalisation); or Type III, consisting of soda-lime-silicate without treatment. The Type I and II containers feature a high hydrolytic resistance compared with those made of Type III glass, which only exhibit moderate hydrolytic resistance. One essential internationally recognised regulatory requirement for demonstrating the sufficient quality and patient safety of glass containers is the combination of composition and performance with a long-established history of safely storing drug formulations.
There is a wide composition range of clear and coloured (amber) glasses that can be used to fulfil the specification for a Type I container, with different amounts of boron oxide, aluminium oxide and alkali oxides. Other oxides, such as alkaline earth oxides and colourants, are added to the glass composition on a case-by-case basis.
“There is a wide composition range of clear and coloured (amber) glasses that can be used to fulfil the specification for a Type I container, with different amounts of boron oxide, aluminium oxide and alkali oxides.”
According to the supplemental industry standard ASTM E438,1 borosilicate glasses with higher amounts of silicon dioxide and boron oxide, along with a more or less alkaline-earth-free glass composition, are described as Type I Class A, which have a low thermal coefficient of expansion (COE) in contrast with glasses with a greater variety of oxides and higher thermal COEs being classified as Type I Class B. Moulded glass and clear and amber “neutral” glasses are other types of borosilicate glass compositions that meet pharmacopeia Type I specifications but fall outside the Type I Class A and Class B groupings of ASTM E438. Therefore, a comfortable situation was reached for the material characterisation of pharmacopeia-defined glass where the labelling of a container as Type I (A) or Type I (B) immediately identifies the container’s composition, relative chemical durability performance and recommended drug formulations to be stored inside.
Complicating the situation is the recently announced update to USP <660> from a composition and performance-based glass-type classification system to a solely performance-based system, which has been in effect since October 1, 2023.2 The USP made this permanent change based on a request from the US FDA to address a temporary shortage of vials to allow pharmaceutical companies to use alternative glass compositions not included in the USP <660>.3 Thus, the updated USP <660> can no longer be used to identify the composition of the glass materials and reinforces the need to characterise the glass composition independently.
To grant a reliable distinction between different Type I glasses, sensitive analytical techniques and a good material database are necessary. SCHOTT Pharma Services uses a combination of X-ray fluorescence (XRF) on ground glass from the container of interest fused to a tablet together with wet chemistry analyses of a glass digestion for boron oxide content. This procedure ensures measurement uncertainties of about 0.5 weight % for silicon and sodium oxides, 0.2% weight % for aluminium and boron oxides and 0.1 weight % or better for the other relevant oxides.
Table 1 compares the results of such composition analyses for four different 2 mL vial types made of tubular glass. The glass used for vial 1 is a typical representative for Type I Class B, while the glasses for vials 2 and 3 fall into the Type I Class A category. In contrast with these clear or flint glasses, additional colouring components are found for amber Type I glasses, as can be seen for vial 4, which contains iron and titanium oxides. Based on these results, all four vials can be assigned to glass compositions published by their manufacturers.4–7
Oxidic component | Vial 1 | Vial 2 | Vial 3 | Vial 4 |
Weight % | Weight % | Weight % | Weight % | |
SiO2 | 75.5 | 80.4 | 80.3 | 70.0 |
B2O3 | 10.5 | 12.5 | 13.0 | 7.9 |
Al2O3 | 5.3 | 2.7 | 2.4 | 5.4 |
Na2O | 7.0 | 4.0 | 3.5 | 6.5 |
K2O | < LoQ | 0.055 | 0.50 | 1.2 |
BaO | < LoQ | < LoQ | < LoQ | 2.1 |
CaO | 1.4 | < 0.05 | < 0.05 | 0.5 |
Fe2O3 | < 0.05 | < 0.05 | < 0.05 | 1.2 |
TiO2 | < LoQ | < LoQ | < LoQ | 4.7 |
Table 1: Chemical composition of different Type I glasses used for different vial types 1–4. The vial was ground and fused to a tablet for semi-quantitative XRF analysis. It was assumed that all elements were present as oxides.
Further information helpful for identifying the dimensional type, such as ISO “R” or non-ISO “R” from ISO 8362-1;8 presence or absence of a blowback in the neck of a glass vial; and, potentially, the specific glass converter, can be generated by documenting the container appearance and dimensional attributes. These data can then be compared with the container drawing or the information provided to the container vendor.
“The “silicone” analysis of syringes and cartridges is mainly focused on the determination of the amount of silicone that could be released.”
The glass composition and the container manufacturing process define which elements can leach into the drug product, and to what extent, over time as a result of drug-container interaction. Vials can be converted from glass tubes (tubular vials) or formed by a press and blow process from a glass gob (moulded vial), which further expands the spectrum of glass compositions.9 Depending on the concentrations, these leachables can be the root cause for pH shifts, formation of particles or precipitations or lead to the initiation and acceleration of drug product degradation.10,11 Therefore, a precise characterisation and quantification of the glass components is also important for ensuring drug stability over time.
COATINGS AND SILICONISATION OF CONTAINERS
Siliconisation is one of the most common treatment methods to modify the behaviour and functionalities of the interior surface of primary packaging containers. Syringes and cartridges require silicone oil on the interior surface as a sliding layer to ensure an acceptable force for plunger movement during injection, while siliconisation of vials can be used to create a hydrophobic surface that can potentially reduce the adsorption of biological molecules or the creeping of lyophilised drug formulations.
A possibly detrimental effect of silicone application is the potential generation of sub-visible particles. The amount of silicone applied for lubrication is significantly higher than that used for a hydrophobic layer on vials. Therefore, the “silicone” analysis of syringes and cartridges is mainly focused on the determination of the amount of silicone that could be released (“total silicone”) by, for example, an ultrasonic extraction with a suitable solvent, such as n-heptane, followed by a quantification of silicon within the extract with atomic absorption spectrometry (GF-AAS).
In the case of vials, a qualitative characterisation of the presence or absence of silicone oil is more relevant in most cases. This question can be addressed by applying time of flight-secondary ion mass spectrometry (ToF-SIMS), which is a very sensitive surface analytical method to identify silicone molecules.12
Applying solid coatings is another method for tailoring properties such as chemical resistance, side-wall adhesion of freeze-dried substances, accurate dosage, pH shift or the prevention of migration of leachates. The thickness of the respective layers is quite small and does not affect the dimensions of the container but is usually sufficient to prevent a direct interaction between the drug product and the container wall. The composition of the layer can vary between totally inorganic, such as silicon dioxide, which is used as a diffusion barrier, and more hydrophobic, such as organosilicon compounds, which are used for lyophilised drug products to prevent creeping of drug formulation during lyophilisation (“fogging”) or liquid formulations in a high-pH regime to minimise chemical glass attack.
The qualitative composition and the thickness of such thin layers can be effectively characterised by ToF-SIMS depth profiling of the interior surface. This profiling is performed in alternating cycles of removing some material from the surface by intensive bombardment with Type A ions (e.g. oxygen), the “cycle of sputtering”, followed by the analysis of the ions that are emitted under slight bombardment with Type B ions (e.g. bismuth), the “cycle of analysis”. This procedure leads to a profile of the intensities of the ions with respect to the sputter time (intensity-sputter-time-profile). Using the sputter rate of a bulk glass, a rough correlation between the sputter time and the “depth” (thickness of the eroded layer) can be achieved.
The developments of positively charged ions derived by the depth profiling from a vial with a thin silicon dioxide layer at the interior surface (SCHOTT Type I plus®) are depicted in Figure 2. Within the first 50 seconds of sputtering time, only the Si+ ion is present with significant intensity before the signals of the typical glass elements (Na+, B+, Al+, Ca+) increase.
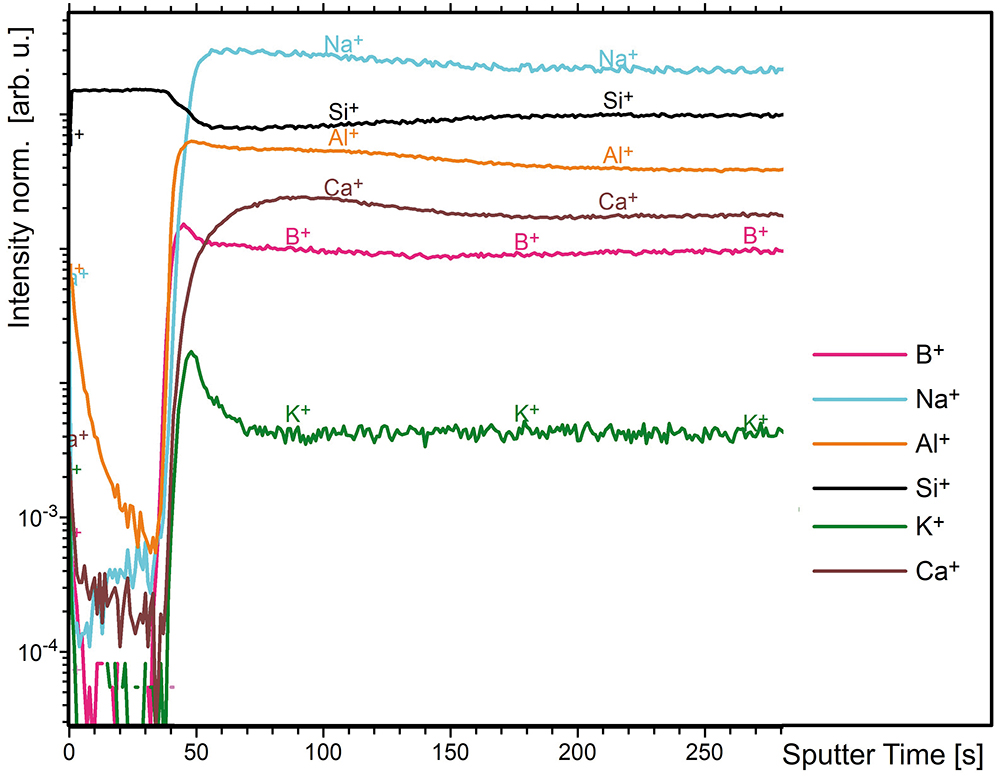
Figure 2: ToF-SIMS intensity-sputter time profiles (positive) from a vial with a thin silicon dioxide layer at the interior surface (SCHOTT Type I plus®).
TREATMENTS AND FURTHER PROCESSING OF CONTAINERS
Further processing of containers is another way to adjust properties, such as improved surface hydrolytic resistance (“dealkalization” by ammonium sulfate treatment),13 strengthening of containers (by ion exchange and washing)14 and reduction of friction (exterior surface coatings).15 Confirmation of these processes being applied to the containers for certificate of analysis verification is straightforward.
Ammonium sulfate treatment is readily proven by visual inspection or direct measurement of sodium sulfate particles present on the inner surface of the treated containers. Ion exchange applied for chemical strengthening can be confirmed through SIMS depth profiling to measure the increased amount of exchanged ion (normally K+ substituting Na+) in a near-surface layer or by optical birefringence methods to assess the depth of the strengthening, as well as by strength testing. The same SIMS method can be used for exterior friction reducing coatings, also in combination with static friction measurements.
For material identification purposes, the confirmation of these treatments after the container has been filled and stored with drug product is similar for ion exchange and surface coatings, but significantly more challenging for ammonium sulfate treatment. This is due to the removal of the direct evidence for treatment (removal of the sulfate species) from the surface during the washing process prior to the container being filled with drug solution, as well as further alteration of the interior glass surface during storage with the drug solution.
“To achieve the successful identification and verification of primary packaging components requires a well-equipped laboratory, capable of conducting a variety of validated wet chemical, chromatographic, spectroscopic and physical measurement methods.”
CONTAINER CLOSURES
Elastomeric components are the second part of the primary packaging system that have contact with the drug product. These are predominantly vial stoppers (serum and lyo), syringe and cartridge plungers, syringe tip caps and cartridge seals. The base material chemical families of the majority of closures used in the pharmaceutical industry are halobutyl-based rubbers, specifically bromo- and chloro-butyls. Other closure system base materials in use are nitrile, butyl, thermoplastic elastomers, styrene-butadiene and polysiloxane. Barrier films and laminates made of ethylene tetrafluorotheylene (ETFE) and polytetrafluoroethylene (PTFE) are often applied to reduce leachables and particulate shedding. Silicone oil or crosslinked silicone coatings are used to improve machineability during assembly.
Due to the large range of compositions, laminates and coatings used for elastomeric closures, identity testing and verification is beyond the scope of USP <381> and Ph Eur 3.2.9. Nevertheless, identity testing is still required, as the corresponding guidance chapter, USP <1381>, states that it is the responsibility of the drug product manufacturer and the component supplier to verify composition and confirm the identity of materials, while Ph Eur 3.2.9. demands that the drug manufacturer requests evidence from the supplier that the composition does not vary and is identical to that used during compatibility testing.
The USP and Eur Ph recommend several possible identification testing methods, such as ash content determination, sulfur determination, specific gravity, chromatography of component extract, attenuated total reflectance Fourier transform infrared spectroscopy (ATR-FTIR), ultraviolet absorption of component extract and FTIR of component extract pyrolysate. The most informative for the purpose of elastomeric component material identification is chromatography of component extract to determine the elastomer’s chemical family and ATR-FTIR to determine the presence or absence of coatings and lubricants, as well as to further confirm the elastomer’s chemical family. Both methods are used for so-called “fingerprint identification”. For ATR-FTIR analysis, stopper samples are prepared to analyse:
- the drug product contact surface
- the non-drug product contact surface
- the bulk interior surface (i.e. cross-section).
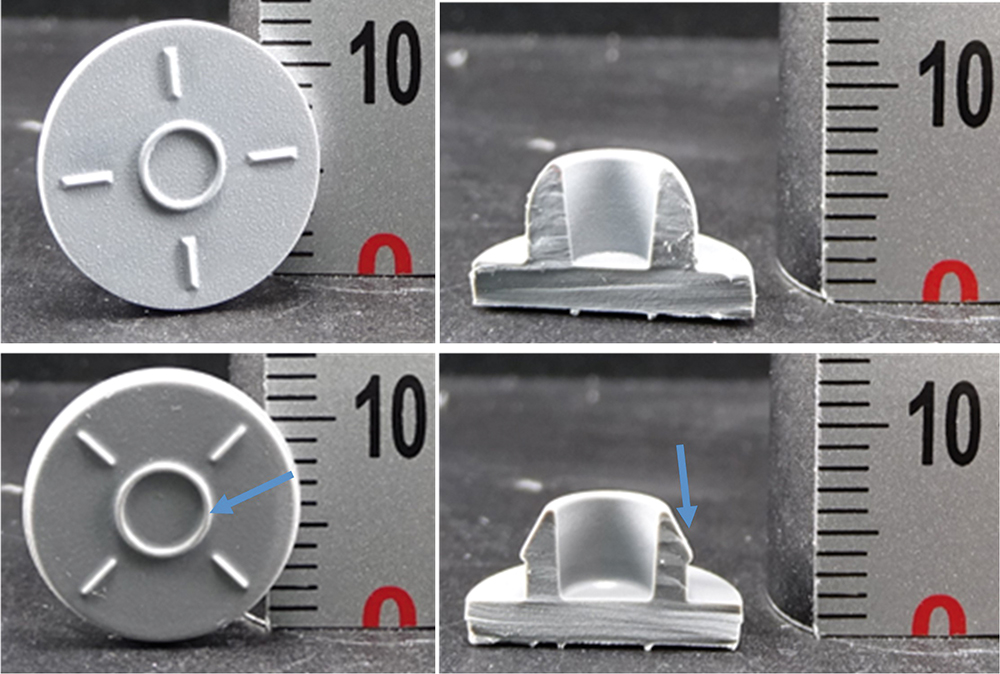
Figure 3: Photographic images of bromobutyl (top) and chlorobutyl (bottom) rubber stoppers. Blue arrows indicate a larger diameter injection ring (bottom left) and presence of a ribbed plug seal (bottom right) of the chlorobutyl rubber stopper being applied in combination with blowback glass vials.
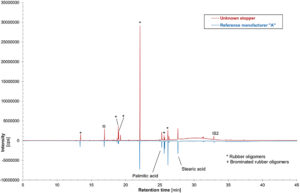
Figure 4: Mirror plotted GC-MS chromatograms of unknown rubber stopper sample extract compared with bromobutyl rubber stopper reference from manufacturer “A” extract. IS: Internal standard “2 fluorobiphenyl”.
A systematic inspection of the visual appearance and geometry is a useful first step in narrowing down and eliminating various stoppers (Figure 3). Determination of the chemical family of the base elastomer is performed by gas chromatography-mass spectrometry (GC-MS) analysis of a stopper extract and an ATR-FTIR assessment of the bulk stopper material. The GC-MS analysis of an unknown serum stopper sample extract is shown in Figure 4 as a mirror plot, showing good accordance with the corresponding analysis of an extract of a reference bromobutyl rubber stopper from manufacturer “A” conducted under the same conditions. An ATR-FTIR analysis of the bulk spectrum of the unknown sample confirms a best-fit match to the same bromobutyl rubber reference stopper cross-section spectrum (Figure 5).
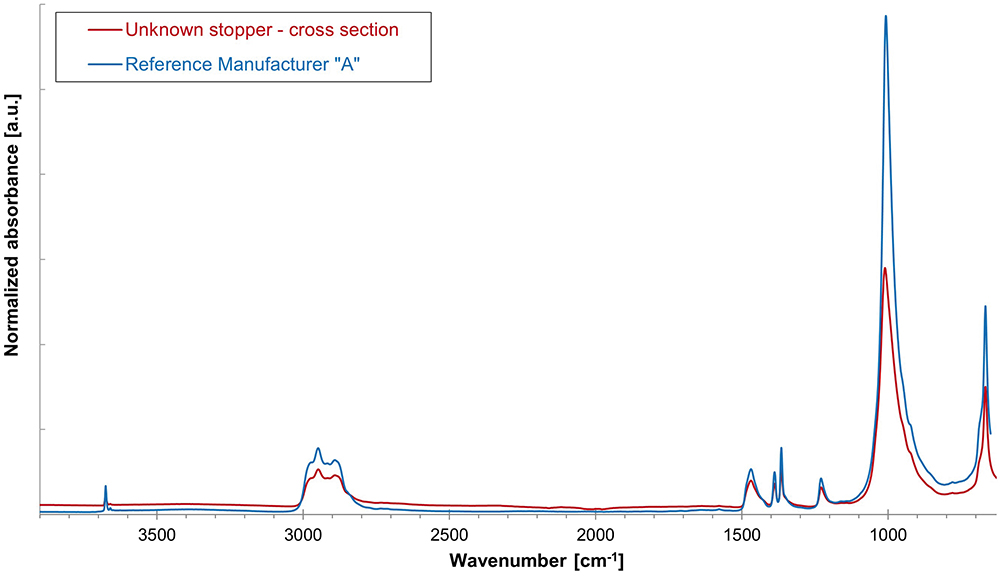
Figure 5: Overlay of cross-section ATR-spectrum of unknown rubber stopper sample compared with ATR cross-section spectrum of bromobutyl rubber stopper reference from manufacturer “A”.
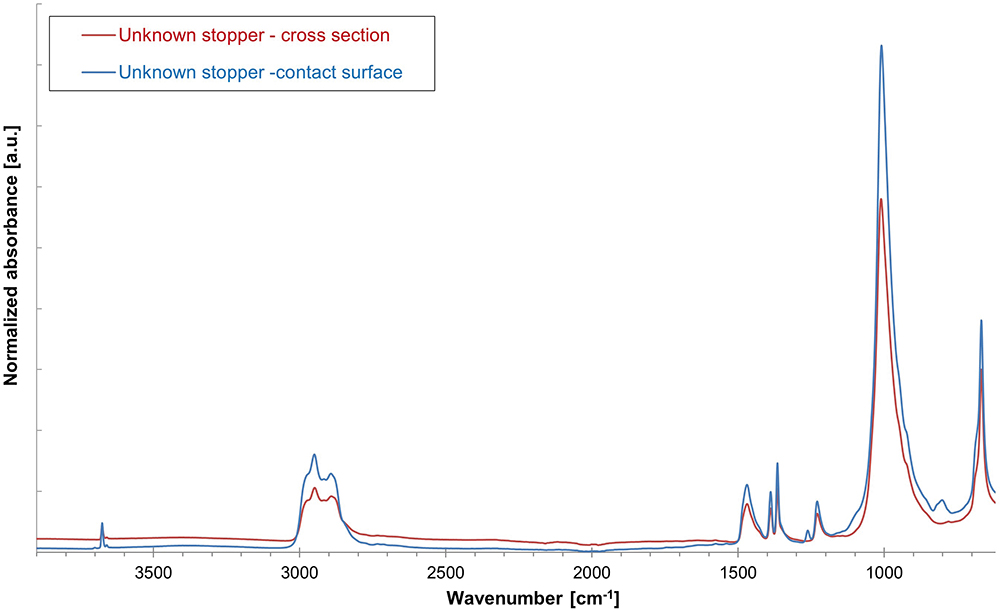
Figure 6: Overlay of drug product contact surface and cross-section ATR-spectra of unknown rubber stopper sample for identification of polymer coating.
The next step is to determine the presence or absence of a barrier coating or film on the elastomer’s face that will be in contact with any drug product and the presence or absence of a siliconising agent. An ATR-FTIR analysis of the unknown stopper sample’s drug product contact surface spectrum versus its bulk spectrum (Figure 6) confirms that a deliberately applied barrier coating or film was not present on the stopper. An ATR-FTIR analysis of the non-drug product contact surface of the unknown sample versus the bulk spectrum (Figure 7) confirms that a deliberately applied siliconising agent was present on the non-drug contact surface, as shown by the presence of an Si-CH3 band at 1260 cm-1.
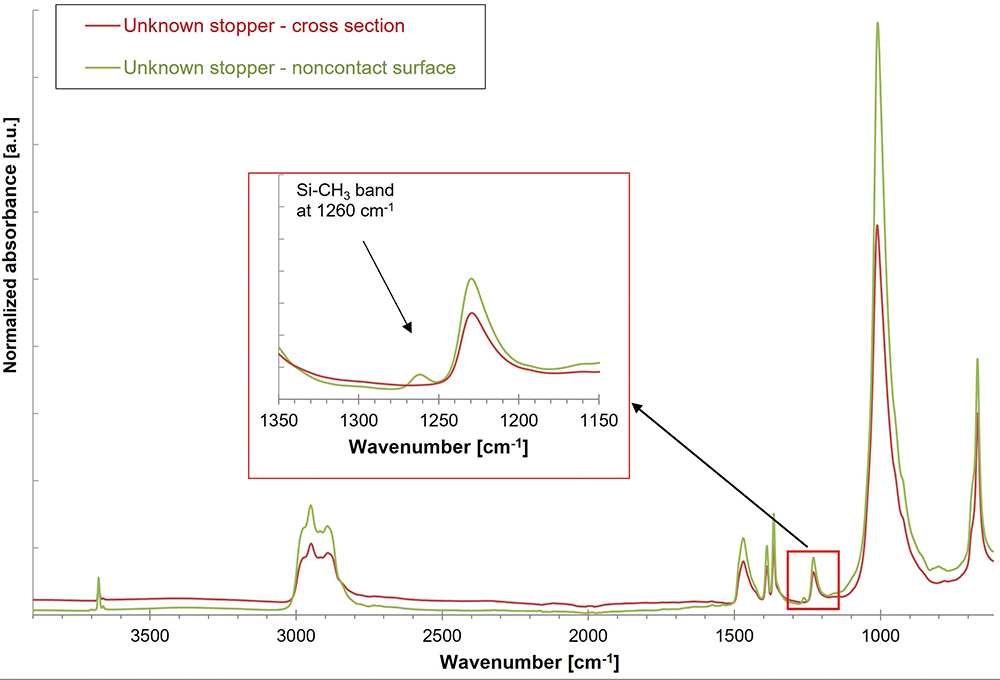
Figure 7: Overlay of drug product contact surface and cross-section ATR-spectra of unknown rubber stopper sample for identification of siliconisation.
POLYMER CONTAINERS
For polymer components, the same methods can be applied for characterisation and identification as for rubber components. As an example, for polymer syringes, the polymer type can be identified by a combination of ATR-FTIR and GC-MS, while the silicone and lubrication can be identified by the methods described in Table 2.
Container property |
Method | ||||
XRF + wet chemistry |
ATR, FTIR | ToF-SIMS | GC-MS | GF-AAS | |
Glass composition | X | ||||
Closure composition |
X | X | |||
Polymer container composition |
X | X | |||
Coating glass / closure |
X | X | |||
Lubricant or silicone layer |
X (indicative) |
X | X | ||
Glass surface treatment |
X |
Table 2: Comprehensive summary of the analytical methods that can be used to characterise the different properties of packaging material components.
SUMMARY
The composition and manufacturer of a given glass container or elastomer closure component can be readily identified and verified using as-received samples or marketed packaging systems by combining various component attributes, such as appearance, colour and dimensions, with the chemical identity of the base material and coating composition, along with any lubrication or surface treatment. To achieve the successful identification and verification of primary packaging components requires a well-equipped laboratory, capable of conducting a variety of validated wet chemical, chromatographic, spectroscopic and physical measurement methods.
A comprehensive allocation of the analytical methods and tools, as well as the properties of packaging material that can be characterised by them, are shown in Table 2. Along with the necessary hardware, experienced staff, including inorganic and organic materials scientists and analytical chemists and physicists, with broad knowledge of primary packaging raw materials, manufacturing and processing methods, as well as a keen understanding of the various regulatory requirements, is essential.
REFERENCES
- “ASTM E438-92(2018) Standard Specification for Glasses in Laboratory Apparatus”. ASTM, Jan 2018.
- “Interim Revision Announcements (IRAs)”. Web Page, USP-NF, accessed Apr 2024.
- “USP is announcing a proposal to modify the glass classifications in General Chapter <660> and to create additional flexibility for packaging and storage requirements in specified monographs”. USP-NF, Jan 2023.
- “FIOLAX® clear”. Data Sheet, SCHOTT, accessed Apr 2024.
- “Pharmaceutical Glass Tubing”. Product Brochure, Corning, 2022.
- “BORO-8330™”. Data Sheet, SCHOTT, accessed Apr 2024.
- “FIOLAX® amber”. Data Sheet, SCHOTT, accessed Apr 2024.
- “ISO 8362-1:2018 Injection containers and accessories – Part 1: Injection vials made of glass tubing”. International Standards Organization, Aug 2018.
- Boltres B, “When Glass meets Pharma: Insights about glass as primary packaging material”. Editio Cantor, Jun 2015.
- Pillai SA et al, “Pharmaceutical Glass Interactions: A Review of Possibilities”. J Pharm Sci & Res, 2016, Vol 8(2), pp 103–111.
- Ogawa T et al, “Comparisons of Aluminum and Silica Elution from Various Glass Vials”. Chem Pharm Bull (Tokyo), 2016, Vol 64(2), pp 150–160.
- Rothhaar U, Haines D, Scheumann V, “Silicone Oil Induced Effects in Pharmaceutical Glass Vials Testing Methods for Visualisation, Identification and Quantification”. Pharm Ind, 2022, Vol 84(1), pp 120–127.
- Crawford A, Zuccato D, Hladik B, “Effective Alternatives for Ammonium Sulfate Treated Pharmaceutical Glass Vials”. Pharm Ind, 2021, Vol 83(1), pp 139–147.
- Bronnbauer C, “Glass Breakage in Pharmaceutical Packaging “. PDA Letter, Nov 2018.
- Roehl H et al, “Risk Mitigation of Drug Shortages-A New Concept for Vials Designed to Improve Fill and Finish Performance”. PDA J Pharm Sci Technol, 2021, Vol 75(2), pp 141–156.