To Issue 170
Citation: de Silva K, Miller E, “Shielded Therapeutics: Could Polymeric Nanoparticles Safeguard a New Generation of Inhaled Drugs?. ONdrugDelivery, Issue 170 (Apr 2025), pp 64–69.
Kamaal de Silva and Ethan Miller provide an overview of the benefits of polymeric nanoparticles in pulmonary drug delivery and their potential to overcome key challenges in respiratory medicine, while summarising the range of pulmonary delivery devices that could benefit from their use.
Polymeric nanoparticles (PNPs) represent a groundbreaking advancement at the cutting edge of pulmonary drug delivery, holding great promise for overcoming key challenges in respiratory medicine. Vital therapeutics, such as small molecules, proteins and nucleic acids, could be encapsulated in engineered nanoscale vehicles to evade the natural defences that protect critical areas of the respiratory system.
PNPs offer significant advantages for drug delivery over the more commonly used lipid nanoparticles (LNPs), which are at a more advanced stage across the industry. For example, Translate Bio’s (Sanofi) MRT5005, an inhaled LNP carrying mRNA administered by nebulisation, is currently in the “Study to Evaluate the Safety & Tolerability of MRT5005 Administered by Nebulization in Adults With Cystic Fibrosis (RESTORE-CF)” Phase I/II trial. Compared with LNPs, PNPs provide greater stability, thus resisting enzymatic degradation and fusion in biological environments.1 They also provide a wider variety of methods for controlled drug release and can be functionalised for targeted delivery. With good biocompatibility, reduced immunogenicity and improved mucus penetration, PNPs could present a more effective alternative to LNPs for pulmonary drug delivery.
BREACHING THE FORTRESS
Drugs intended to have systemic effects must reach the alveoli – the deep lung region with a huge surface area totalling an estimated 140 m2 and a rich blood supply, both of which facilitate gas exchange. To protect this vital function, the lungs have a unique and multilayered defence system that filters out any foreign invaders. Particles larger than 5 μm are subject to the mucociliary escalator; the self-clearing mechanism consisting of the mucous layer and cilia underneath, as shown in Figure 1.
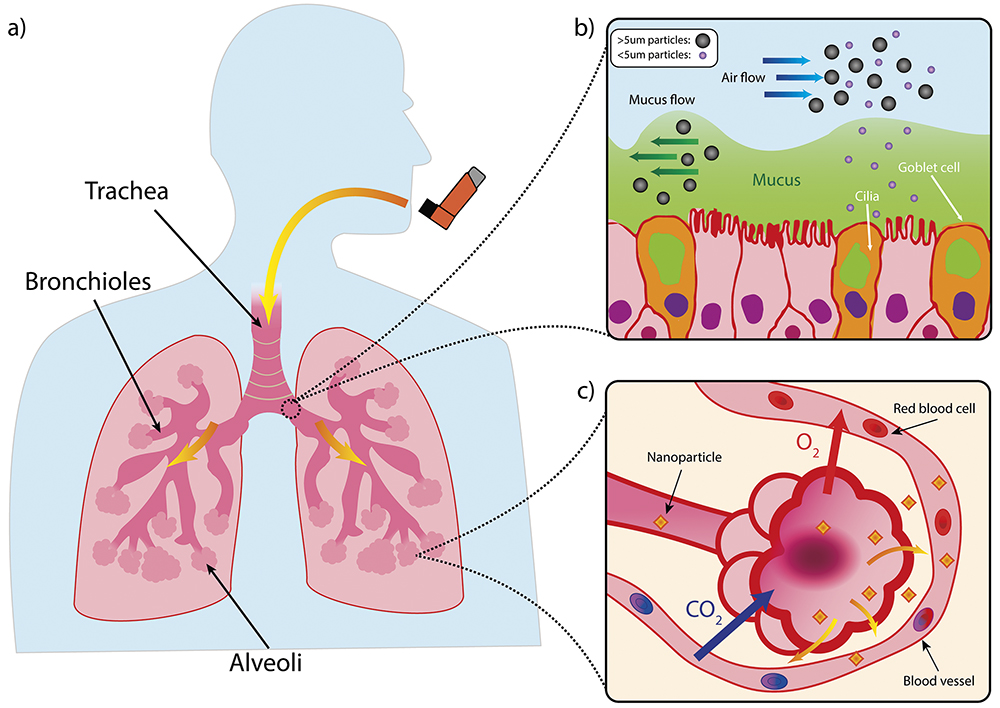
Figure 1: Structure of lung and mucociliary escalator. a) Simplified illustration of a lung. b) Illustration of mucociliary escalator clearing larger particles from the airways. c) Diagram of alveoli with large surface area to facilitate gaseous exchange but also provide route for systemic delivery of nanoparticles via the blood stream.
While technically they may be able to “reach” the alveolar region due to their size, submicron particles (i.e. <1,000 nm-sized particles) have such low inertia that they would simply be exhaled.2 PNPs therefore require a supporting medium to achieve particle sizes between 1 μm and 5 μm, which is the correct range for deposition in the deep lung via sedimentation.3 The closer the particles can get to the smaller end of this scale, the better for alveolar access.4
“TO ACHIEVE THE RIGHT PARTICLE SIZE FOR THE APPLICATION, THE INHALATION DEVICE USED FOR DELIVERY OF PNPS MUST BE CAREFULLY CHOSEN OR DESIGNED TO ENSURE THAT THE DELIVERY OF THE THERAPEUTIC DOES NOT FAIL AT THE FINAL HURDLE.”
To achieve this, inhalation devices are therefore designed to give a high fine particle fraction (FPF), which is the percentage of aerosolised particles <5 μm, and a low mass median aerodynamic diameter (MMAD); the representative diameter below which 50% of the particle population of an aerosol lies. PNPs could also have classical respiratory applications where the target may be the bronchi and bronchioles themselves, for example, facilitating the delivery of bronchodilators or corticosteroids.
To achieve the right particle size for the application, the inhalation device used for delivery of PNPs must be carefully chosen or designed to ensure that the delivery of the therapeutic does not fail at the final hurdle. Table 1 shows some potential options for PNP-based delivery devices.
Technology | MMAD (μm) | Suitability for Systemic Delivery | Suitability for PNP Delivery |
Pressurised metered dose inhalers (pMDIs) | 1.2–8 | The technology is suboptimal due to generally larger-sized particles, inconsistent lung deposition rates (8–53%) and high oropharyngeal loss5 | The high-pressure storage environment (~5.6 barg)6 and high shear forces during actuation could disrupt PNPs |
Dry powder inhalers (DPIs) | 1.8–4.8 | Particle sizes can be engineered to an optimal size, as is the case for MannKind’s (CT, US) Afrezza (insulin) with an MMAD of 2.5 μm,7 but lung deposition rates (7–69%) are highly dependent on the patient’s inspiratory flow rate5 |
High suitability, inherent capability to avoid nanoparticle aggregation and enhance stability3 |
Soft mist inhalers (SMIs) | 3.7 | Produces a slow-moving mist with a FPF of 60% and lung deposition rate of 39–67%, excellent for deep lung penetration6 | Excellent suitability, and Miao H et al (2023) showed that lipid nanoparticles, which are weaker against shear, can survive atomisation8 |
Thermal vaporisation (Ferrer’s Staccato) | 2 | Generates an ultrafine aerosol with an FPF of 90%, enabling rapid alveolar absorption, without using any excipients9 – currently US FDA approved for the delivery of loxapine, an antipsychotic medication | The heat generated during vaporisation is likely to degrade temperature-sensitive PNPs |
Jet and ultrasonic nebulisers | 2.1–6.8 | Capable of providing small droplets,10–12 but low lung deposition rates (5–13%)13 mean that a lot of the drug will be wasted, and there may be a wide range in performance between device manufacturers | High shear forces can disrupt liposomes4 but PNPs might be more resistant |
Vibrating mesh nebulisers | 3–5 | Generates a uniform, slow-moving aerosol with high FPFs of 50–70%14 for deep lung penetration | Demonstrated excellent suitability for delivering vesicles such as liposomes, and can even function to break up aggregates4 |
Table 1: Pulmonary delivery devices and their suitability for systemic and PNP delivery.
Once the device has delivered it to its intended location in the lung, the PNP can then carry out its key functions, which could be a mixture of:
- Protecting sensitive therapeutics, such as nucleic acids and proteins, from degradation in the harsh respiratory environment to enhance their delivery efficiency. Respiratory mucus contains nucleases and proteases that would quickly degrade them via enzymatic attack.15
- Targeting specific cell types within the lung (e.g. tumour cells) using ligands placed on the shell, for example, antibodies or peptides.3
- Providing transport mechanisms for biologics that, if unprotected, would be immobilised by mucin fibres – PNPs with muco-inert coatings, such as polyethylene glycol (PEG), can reduce adhesion for better diffusion through the mucous mesh.16
- Evading phagocytosis by macrophages by engineering the shell or surrounding matrix of the PNP, thus sidestepping one of the primary clearing functions of the innate immune system. For most microspheres, engulfment via macrophage is something to be avoided; however, there are some cases, such as vaccines, where triggering a macrophage response is the aim of the therapy.17
- Improving drug uptake into the cells via endocytosis-based pathways.3
- Translocating from the alveoli to the systemic circulation, which, for certain drugs, could remove the need for intravenous administration.3
NANOSCALE BLUEPRINTS
Figure 2 provides some examples of the wide variety of different PNPs that can be used in pulmonary delivery. Much of the literature categorises PNPs into two types:19, 20
- Nanocapsules: The drug is usually dissolved in an aqueous or oily reservoir, the release of which is controlled by a polymeric membrane wrapped around it.
- Nanospheres: A continuous matrix system where the drug is retained inside or adsorbed onto its surface.
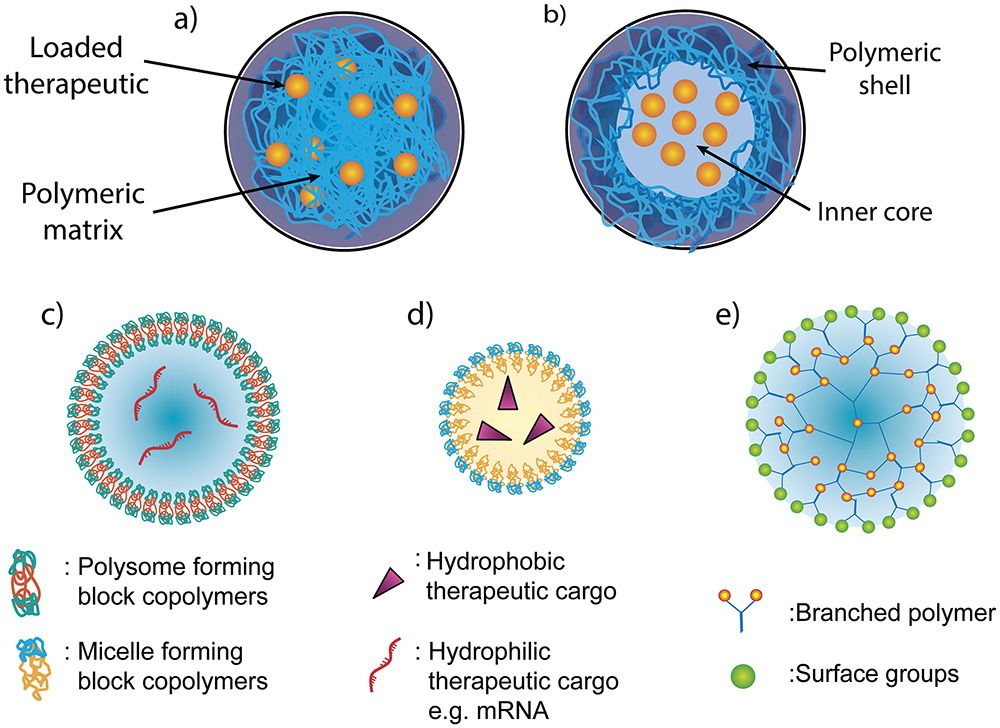
Figure 2: Different structures of PNPs. The main categories of PNP for pulmonary delivery are (a) nanospheres and (b) nanocapsules. Nanoparticles can also exist in various shapes, including (c) polymersomes, (d) polymer micelles and (e) dendrimers.
Within the above categories, PNPs can be divided further into specific shapes, such as:20
- Polymersomes: Artificial hollow spheres (vesicles) with membranes built from amphiphilic block copolymers (e.g. PEG), which are comparable to liposomes – vesicles formed from naturally occurring lipids.
- Polymer Micelle: Also built from amphiphilic block copolymers that instead form nanospheres with a hydrophilic core and hydrophobic coating.
- Dendrimers: Hyperbranched polymers, such as polyethyleneimine (PEI), with complex 3D architectures. Active functional groups on the outer surface can be used to conjugate biomolecules and contrast agents for imaging. They are mostly used for the delivery of nucleic acids or small molecules, which can be enclosed within.
PNPs can be constructed from a wide range of polymer materials, examples of which are shown in Table 2, along with potential pulmonary applications that are currently under research.
Type | Polymer | Mechanisms of Action | Potential Applications |
Natural | Chitosan |
|
|
Gelatin |
|
|
|
Synthetic | PLGA |
|
|
PLA |
|
|
|
PEtOx |
|
|
|
PCL |
|
|
|
PEI |
|
|
|
Polystyrene |
|
|
|
PVA |
|
|
Table 2: PNPs with pulmonary applications.21
SEMI-AUTOMATED ASSEMBLY
multitude of different processes exist to create the PNPs in Table 2, which either involve monomer polymerisation as part of the process or the dispersion of pre-formed polymers. Polymerisation techniques, used for polymers such as poly(lactic-co-glycolic acid) (PLGA), polylactic acid (PLA) and polycaprolactone (PCL), usually involve the formation of an emulsion, which can go on to self-assemble into nanoparticles.
When using pre-formed polymers to form PNPs, such as polystyrene, polyvinylcarbazole (PVK) and poly(methyl methacrylate) (PMMA), a solvent is normally used, and precipitation of nanoparticles can be induced by removal of the solvent by evaporation/diffusion, adding salt or desolvation. In both categories of PNP formation, surfactants, such as polyvinyl alcohol (PVA) or crosslinkers, can also be used to control size and prevent aggregation, but need to be eliminated from the final product.18
Compared with the LNP processes used for covid-19 vaccines, which used microfluidics at small scale and were scaled up by using confined impinging jet mixers,22 more steps are required to synthesise PNPs and, therefore, much more research is required to optimise them for mass production. PLGA and PLA are promising candidates for scalability, as these materials are widely used across the industry. Operti et al (2022) demonstrated an encapsulation efficiency of 80% for PLGA nanoparticles encapsulating celecoxib using sonication23 and, in 2024, Maurizii et al demonstrated 90% encapsulation efficiency for chitosan nanoparticles using microfluidic-assisted ionotropic gelation.24
“INTERACTIONS BETWEEN PNPS AND BIOLOGICAL BARRIERS DEPEND STRONGLY ON THE PHYSICOCHEMICAL CHARACTERISTICS OF THE NANOPARTICLE SURFACE, SIZE, SHAPE AND HYDROPHOBICITY.”
FINE-TUNED FUNCTION
Interactions between PNPs and biological barriers depend strongly on the physicochemical characteristics of the nanoparticle surface, size, shape and hydrophobicity. One associated challenge is the electrostatic and hydrophobic interactions between PNPs and the mucus barrier, as well as exopolysaccharide biofilms present in the pulmonary tracts.25 PEGylation of the PNP surface gives it a sufficiently hydrophilic surface and improves its ability to penetrate mucus and biofilm layers, enhancing drug delivery.25 This technique was successfully applied to polyethylenimine-graft-poly(ethylene glycol) (PEI-g-PEG) nanoparticles acting as a nanocarrier of mRNA for gene transfection specifically targeting lung tissue in mice models.26 Notably, these functionalised PNPs could be stored in lyophilised form at -20°C for over four months, without a measurable change in particle size or transfection activity, demonstrating exceptional stability.26 Additionally, recent studies have even indicated that replacing PEG functionalisation of nanoparticles with zwitterionic polymers may also improve nebuliser stability and delivery efficacy, as well as reduce aggregation.27
PNPs can also be functionalised to exist more stably in the micro-environment of the lung. PNPs responsive to reactive oxygen species (ROS) have emerged as a promising platform for pulmonary drug delivery, offering targeted and efficient therapeutic modulation of lung injury microenvironments. When lung tissue is damaged, cells release large amounts of ROS into the lung microenvironment, which can lead to oxidative damage and increase inflammation and tissue injury.
In a 2022 study by Muhammad et al, this ROS response was used to hallmark lung injury and trigger therapeutic release from PNPs.28 By incorporating ROS-sensitive linkages within the polymer matrix, PNPs loaded with anti-inflammatory dexamethasone can achieve more targeted drug release, improving local anti-inflammatory responses, minimising systemic exposure and maximising therapeutic efficacy. Compared with free dexamethasone, PNPs exhibit superior pharmacokinetics and biodistribution, maximising drug retention in pulmonary tissues while minimising off-target effects. This study highlights the promise of ROS-responsive PNPs as a game changer for treating lung diseases driven by oxidative stress, such as acute lung injury (ALI) and chronic obstructive pulmonary disease (COPD), opening the door for future breakthroughs in pulmonary nanomedicine.
“NANOPARTICLES CAN BE ENGINEERED FOR INHALATION DELIVERY, ENSURING DEEP LUNG PENETRATION AND PROLONGED THERAPEUTIC ACTION.”
In pulmonary diseases such as COPD, pneumonia and ALI, thermally triggered PNPs can enhance drug retention in the lungs while reducing off-target toxicity. Additionally, these nanoparticles can be engineered for inhalation, ensuring deep lung penetration and prolonged therapeutic action.
A MINIATURE FUTURE
PNPs represent a paradigm shift in pulmonary drug delivery, overcoming physiological barriers through rational design. Their stability, modularity and compatibility with inhalation devices position them as versatile platforms for treating a huge range of conditions. Future studies must address scalability and toxicity challenges and continue to explore combinatorial therapies that harness the unique physiochemical properties of PNPs. In the future, it is likely that PNPs will play a central role in the evolution of inhalation technology.
REFERENCES
- Danhier F et al, “PLGA-based nanoparticles: An overview of biomedical applications”. J Control Release, 2012, Vol 161 (2), pp 505–522.
- Maloney Norcross SE et al, “Biopolymeric Inhalable Dry Powders for Pulmonary Drug Delivery”. Pharmaceuticals, 2024, Vol 17 (12), art 1628.
- Chan HW et al, “Inhalable Nanoparticle-based Dry Powder Formulations for Respiratory Diseases: Challenges and Strategies for Translational Research”. AAPS PharmSciTech, 2023, Vol 24 (4), art 98.
- Rudokas M et al, “Liposome Delivery Systems for Inhalation: A Critical Review Highlighting Formulation Issues and Anticancer Applications”. Med Princ Pract, 2016, Vol 25 (Suppl 2), pp 60–72.
- Baloira A et al, “Lung Deposition and Inspiratory Flow Rate in Patients with Chronic Obstructive Pulmonary Disease Using Different Inhalation Devices: A Systematic Literature Review and Expert Opinion”. Int J Chron Obstruct Pulmon Dis, 2021, Vol 16, pp 1021–1033.
- “ZEPHEX® 134a Medical Propellant” Propellant, Product Brochure. Koura Global, Mar 2025.
- Greene SF et al, “Long-Term Nonclinical Pulmonary Safety Assessment of Afrezza, a Novel Insulin Inhalation Powder”. Toxicol Pathol, 2021, Vol 49 (2), pp 334–348.
- Miao H et al, “Optimization of formulation and atomization of lipid nanoparticles for the inhalation of mRNA”. Int J Pharm, 2023, Vol 640, art 123050.
- Noymer P et al, “The Staccato® System : Inhaler Design Characteristics for Rapid Treatment of CNS Disorders”. Proc Respiratory Drug Delivery, 2010. Vol 1, pp 11–20.
- Adorni G et al, “Aerosolization Performance of Jet Nebulizers and Biopharmaceutical Aspects”. Pharmaceutics, 2019, Vol 11 (8), art 406.
- Elhissi AMA et al, “Delivery of liposomes generated from proliposomes using air-jet,ultrasonic, and vibrating-mesh nebulisers”. J Drug Deliv Sci Technol, 2005, Vol 15 (4), pp 261–265.
- Katial RK et al, “Comparison of three commercial ultrasonic nebulizers”. Ann Allergy Asthma Immunol, 2000, Vol 84 (2), pp 255–261.
- Ari A, Fink JB, “Delivered dose with jet and mesh nebulisers during spontaneous breathing, noninvasive ventilation and mechanical ventilation using adult lung models”. ERJ Open Res. 2021, Vol 7 (3), pp 00027–02021.
- Waldrep JC et al, “Comparative Analysis of Methods to Measure Aerosols Generated by a Vibrating Mesh Nebulizer”. J Aerosol Med, 2007, Vol 20 (3), pp 310–319.
- Mendes BB et al, “Nanodelivery of nucleic acids”. Nat Rev Methods Primers, 2022, Vol 2 (1), pp 1–21.
- Schuster BS et al, “Nanoparticle diffusion in respiratory mucus from humans without lung disease”. Biomaterials, 2013, Vol 34 (13), pp 3439–3446.
- Champion JA et al, “Role of Particle Size in Phagocytosis of Polymeric Microspheres”. Pharm Res, 2008, Vol 25 (8), pp 1815–1821.
- Zielińska A et al, “Polymeric Nanoparticles: Production, Characterization, Toxicology and Ecotoxicology”. Molecules, 2020, Vol 25 (16), art 3731.
- Crucho CIC et al, “Polymeric nanoparticles: A study on the preparation variables and characterization methods”. Mater Sci Eng C, 2017, Vol 80, pp 771–784.
- Mitchell MJ et al, “Engineering precision nanoparticles for drug delivery”. Nat Rev Drug Discov, 2021, Vol 20 (2), pp 101–124.
- Cojocaru E et al, “Nanoparticle- Based Drug Delivery Systems in Inhaled Therapy: Improving Respiratory Medicine”. Pharmaceuticals (Basel), 2024, Vol 17 (8), art 1059.
- Subraveti SN et al, “Synthesizing Lipid Nanoparticles by Turbulent Flow in Confined Impinging Jet Mixers”. J Vis Exp, 2024, Vol 210, art e67047.
- Operti MC et al, “Industrial Scale Manufacturing and Downstream Processing of PLGA-Based Nanomedicines Suitable for Fully Continuous Operation”. Pharmaceutics, 2022, Vol 14 (2), art 276.
- Maurizii G et al, “Non-invasive peptides delivery using chitosan nanoparticles assembled via scalable microfluidic technology”. Carbohydr Polym Technol Appl, 2024, Vol 7, art 100424.
- Huang Z et al, “Nanoparticlemediated pulmonary drug delivery: state of the art towards efficient treatment of recalcitrant respiratory tract bacterial infections”. Drug Deliv Transl Res, 2021, Vol 11 (4), pp 1634–1654.
- Ke X et al, “Surface-Functionalized PEGylated Nanoparticles Deliver Messenger RNA to Pulmonary Immune Cells”. ACS Appl Mater Interfaces, 2020, Vol 12 (32), pp 35835–35844.
- Jiang AY et al, “Zwitterionic Polymer-Functionalized Lipid Nanoparticles for the Nebulized Delivery of mRNA”. J Am Chem Soc, Vol 146(47), pp 32567–32574.
- Muhammad W et al, “ROSresponsive polymer nanoparticles with enhanced loading of dexamethasone effectively modulate the lung injury microenvironment”. Acta Biomater, 2022, Vol 148, pp 258–270.