Citation: Smith S, Scanlan E, Colavita P, “Surface-Mediated Aggregation – Control of the Liquid-Solid Interfacial Stress”. ONdrugDelivery, Issue 117 (Mar 2021), pp 46–50.
Shane Smith, Eoin Scanlan and Paula Colavita discuss how the company’s technology has evolved to create a stable and biocompatible coating that maintains device performance and safety.
Developability of biologics is often associated with a number of major stumbling blocks – mostly related to potency, safety and manufacturability – that can significantly hinder their commercial viability. Protein stability is one developability obstacle that needs to be resolved during clinical development to avoid costly late-stage safety issues. Proteins are innately susceptible to instability due to their complex molecular architecture and their intrinsic sensitivity to interfacial stresses (air-liquid, liquid-solid and liquid liquid) encountered during manufacturing, storage and administration only exacerbates the problem.
INTERFACE STRESS AND CLINICAL EVIDENCE
The problem with interfacial stresses is that they induce instability and, consequently, can lead to the formation of aggregated proteins. Simplistically, aggregation involves some measure of protein conformational change away from the native folded structure that permits a protein-protein interaction chain reaction, leading to the growth of protein aggregates with size in the subvisible and even visible ranges (Figure 1).
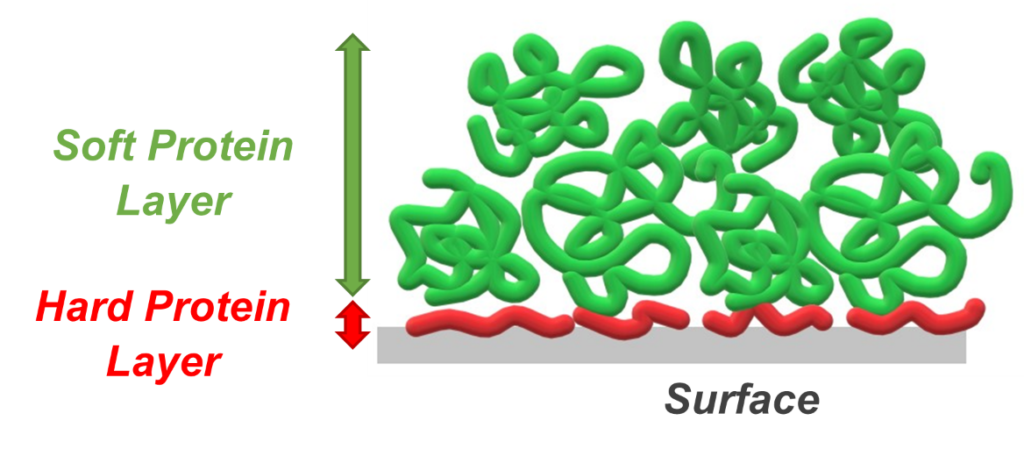
Figure 1: Schematic of typical protein adsorbate structures formed at a generic solid surface.
Formation of aggregates may elicit an immune response; therefore, systematic evaluations of aggregate formation are routinely performed during product development. For example, during formulation development, the primary packaging container and protein of interest are incubated together for various periods of time. The protein and particle concentration is calculated before and after the incubation to determine the container/drug stability profile. Another undesirable consequence of liquid-solid interfacial interaction (adsorption and aggregate formation) phenomena is the reduction in formulation potency and, ultimately, therapeutic efficacy.
“Surface-mediated unfolding (liquid-solid interface) is a significant challenge for drug delivery device manufacturers considering the intrinsic propensity of some materials to adsorb/desorb proteins.”
Both the US FDA and the EMA are becoming increasingly vigilant towards protein immunogenicity and have published guidelines for industry.1 Within these guidelines, the FDA has detailed clinical consequences in efficacy and safety that can result from reduced stability and pose a major risk for product failure and recalls.
The development of anti-drug antibodies as an immune response following the administration of protein therapeutics is a very serious and growing concern among manufacturers, physicians, informed patients and regulatory bodies alike. For example, insulin is administered frequently by diabetic patients and continues to elicit an antibody response regardless of purity and origin.2 Similarly, beta interferon, administered regularly for the treatment of multiple sclerosis, triggers neutralising antibodies associated with a loss of bioactivity and decreased clinical efficacy of the drug.3 At the extreme end of the spectrum, the production of antibodies against erythropoietin can result in very serious adverse side effects, including an almost total cessation of the production of red blood cells.4
LIQUID-SOLID INTERFACIAL STRESS IN STORAGE CONTAINERS
For more than two decades, prefillable syringes (PFSs) have been increasingly adopted by pharmaceutical companies for their increased safety, improved dosing accuracy and convenience for patient and medical workers. However, surface-mediated unfolding (liquid-solid interface) is a significant challenge for drug delivery device manufacturers considering the intrinsic propensity of some materials to adsorb/desorb proteins. The current understanding is that aggregation from the surface may occur as a result of (i) misfolding on the surface then desorption as aggregates and/or (ii) misfolding rapidly upon desorption in solution. Regardless of the mechanism, absorption of proteins at interfaces has been empirically implicated in aggregate formation.5-7 Cyclic olefin polymer (COP) PFSs are well-known for their advantages over glass, including the flexible moulding design for various drug delivery devices and reduced risk of breakage. In fact, the number of biopharmaceuticals supplied in COP PFSs is increasing dramatically. Although COP has, on occasions, displayed a reduced propensity to adsorb and aggregate protein compared with glass, it has been shown that it is not always the case.
“Surfactants mitigate aggregation mediated by liquid-solid interactions and are believed to function through competing access to surfaces. Unlike ionic surfactants, non-ionic surfactants usually do not denature proteins and therefore remain the premier choice for biologic formulations.”
For example, a study comparing the stability of various proteins demonstrated that COP and glass induced comparable percentages of cytokine adsorption formation at chilled temperatures, but at accelerated temperatures slightly more protein adsorbed to COP.8 However, in the same study, a monoclonal antibody (mAb) tested had negligible adsorption with COP, unlike the glass after prolonged storage.8 Therefore, the magnitude of the adsorption/aggregation is protein unique and requires product specific stability profiling with the intended primary packaging material. Characterised desorbed proteins from COP and glass have been reported to be a mixture of monomers, dimers and even small amounts of high molecular weight aggregates.8-10
Protein aggregation can also be mediated by normal syringe operation stresses.10 For example, it was revealed that during ejection, sweeping of the inner barrel surface by the plunger head resulted in displacement of adsorbed proteins and particle generation. It was concluded that micron aggregate concentration in ejected solutions generally increased with increasing density of adsorbed proteins. Although the COP syringes tested exhibited lower aggregate concentrations compared with glass, it was attributed to lower adsorption densities, which we know is not a general trend for proteins.
A major concern is that biologics require higher therapeutic doses – on average 0.1 mg to 3 mg per kg of patient body weight – and therefore require substantially more concentrated formulations (which can exacerbate adsorption/aggregation). This is intensified further by the push for intravenous administration of volumes that are small enough for patient convenience and comfort. To ameliorate these problems and offer an improved safety profile for patients, clearly there is a need for a new suite of technologies to minimise and control protein adsorption and aggregation.
SURFACTANTS – THE DANGERS OF POLYSORBATES
“Using the success of sugars as a foundation to build upon, Glycome BioPharma has created the next generation of carbohydrate mediated protein stabilisation technology by covalently immobilising sugars onto protein-container interfaces.”
As their name implies, surfactants have been adopted as a strategy to alleviate unwanted protein therapeutic adsorption, however, they possess some significant caveats. Surfactants mitigate aggregation mediated by liquid-solid interactions and are believed to function through competing access to surfaces.11 Unlike ionic surfactants, non-ionic surfactants usually do not denature proteins and therefore remain the premier choice for biologic formulations. Yet, adequate stabilisation is reliant on the selection of surfactant and its formulation concentration, as well as on a variety of factors, including a biologic’s concentration, other excipients, container type, and headspace and test methodology.
In biotherapeutics, polysorbates are among the most commonly used functional excipients. Surprisingly, these polyoxyethylene-based surfactants (polysorbate 20 and polysorbate 80) spontaneously autoxidise, yielding reactive peroxides, which, in turn, produce oxidative changes in the protein that consequently induces immunogenicity.12 Furthermore, the rate of oxidative damage is not limited to the concentration of reactive oxidative species, the concentration of the therapeutic protein itself contributes also. As discussed prior, the problem is exacerbated further by the trend towards more concentrated formulations.
More concerningly, polysorbates are attracting attention as an inducer of anaphylaxis. Anaphylaxis is a serious allergic reaction that is connected with the administration of some therapeutic proteins, and as proteins themselves are often inducers of anaphylaxis, naively few, if any, attempts are made to differentiate them from excipients.13 However, clinical evidence is mounting that supports excipient-related anaphylaxis. For example, two patients receiving mAb omalizumab had significant anaphylaxis reactions and subsequent intradermal testing linked it to polysorbate14 and skin prick examinations of erythropoietin-reported hypersensitivity with polysorbate formulations only.15
With polysorbates being formulated with more than 70% of mAbs and other biologics, it is not surprising that rituximab, ofatumumab, obinutuzumab, trastuzumab, tocilizumab, infliximab, adalimumab and omalizumab, which all contain polysorbate surfactant, have reported drug hypersensitivity in patients (Table 1).16 Polysorbates’ application in preventing unwanted immunogenicity can certainly be contradictory, given that immunogenic aggregates are prevented by the addition of surfactants and surfactant-generated peroxides cause an increase in unwanted protein immunogenicity.
Product | API Concentration (mg/mL) |
Polysorbate Conc. (mg/mL) |
Hypersensitivity Reactions |
Rituximab (Rituxan®) | 10.000–500.000 | 0.700 | 5–10% |
Ofatumumab (Arzerra®) | 20.000 | 0.200 | 2% |
Trastuzumab (Herceptin®) | 22.000 | 0.090 | 0.6–5% |
Tocilizumab (Actemra®) | 80.000–400.000 | 0.500 | 0.1–0.7% |
Infliximab (Remicade®) | 100.000 | 0.500 | 1% |
Adalimumab (Humira®) | 40.000 | 0.800 | 1% |
Omalizumab (Xolair®) | 202.500 | 0.500 | 0.9–0.2% |
Table 1: Examples of case reports of hypersensitivity in biologics formulated with polysorbates.16
IMMOBILISED SUGAR STABILISERS – A BETTER SOLUTION
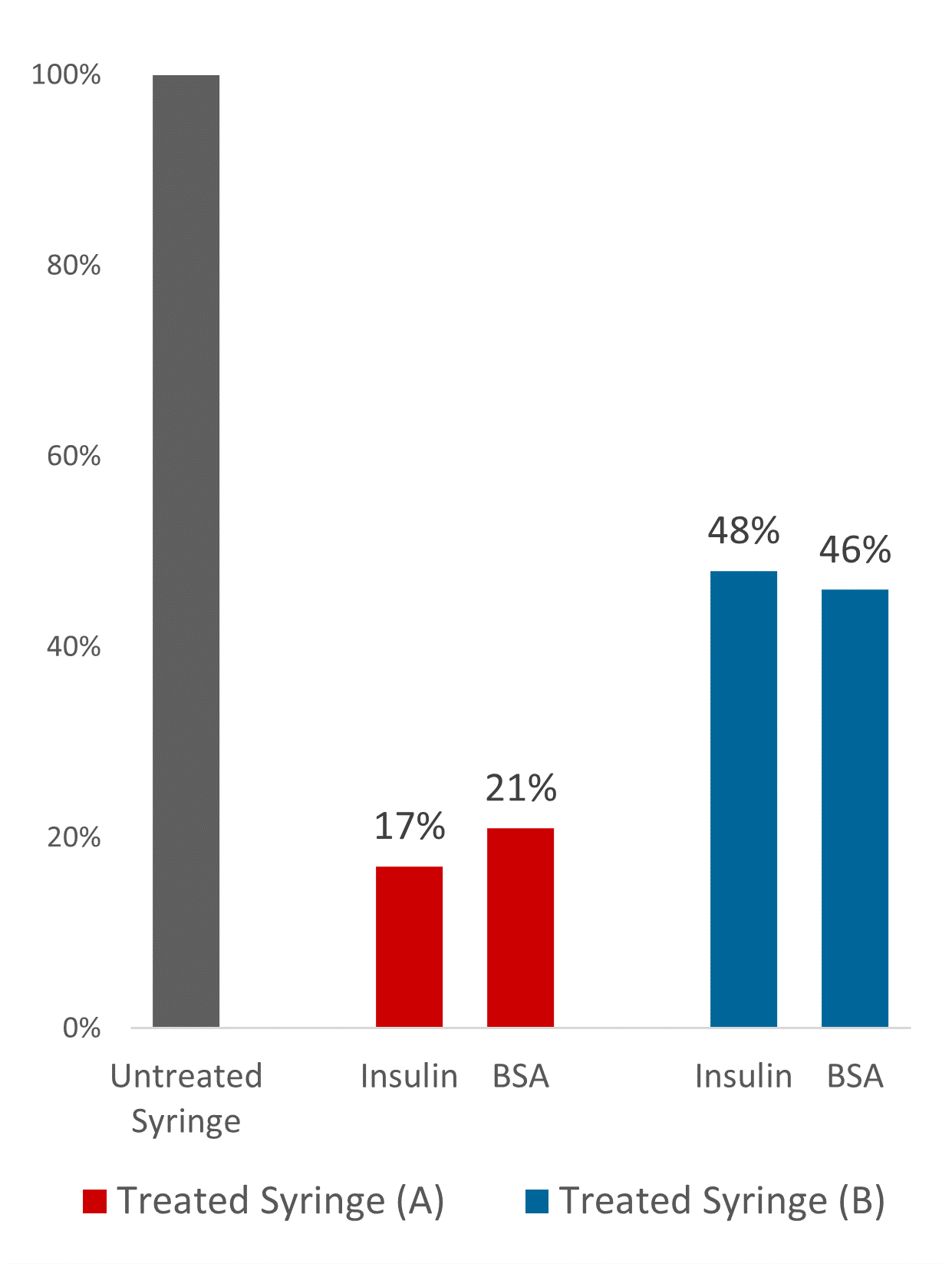
Figure 2: Reduction in adsorbed protein on commercially available olefin PFSs.
Sugar-based excipients have been used for decades to stabilise proteins and consequently have received a reliable reputation. Although, sugars function as excellent stabilisers for therapeutic proteins, it is acknowledged that because each biotherapeutic presents unique formulation challenges, no one stabiliser works best for all molecules. For example, sugars can spontaneously covalently attach to the biologics (glycation), affecting the protein’s structure and function. For instance, it has been reported that glycation of immunoglobulin G2 (IgG2) mAb can occur with sucrose formulations. Analysis mapped the glycation sites to 10 lysine residues throughout the mAb.17 Furthermore, a consideration for formulators is that sugars can detach during freeze-thawing resulting in poor stabilisation efficiency. Freezing and drying are routinely practised for long-term stabilisation and storage of therapeutic proteins. Sucrose is the only recognised sugar to maintain the critical amorphous stabilising state during freezing and thawing.
Using the success of sugars as a foundation to build upon, Glycome BioPharma has created the next generation of carbohydrate-mediated protein stabilisation technology by covalently immobilising sugars onto protein-container interfaces. This commercially validated platform uses only native human sugars (non-derivatised or non-synthetic), similar to those found in the extracellular region of the cell membrane, known as the glycocalyx, and contributes to the steric repulsion that prevents undesirable non-specific adhesion of other molecules and cells.
Researchers have alluded to the opportunities of using glycan-modified surfaces in biomedicine, yet commercialisation proves challenging due to use complex or unsafe functionalisation methodologies. For instance, dextran physisorption has been shown to reduce albumin and plasma adsorption by 90% and 70% at polyethylene surfaces. However, mere physisorption can result in potential leachates, while the dextran modifications required to enhance surface immobilisation involved potentially harmful reactants.18 Additional examples of these commercialisation barriers can be found in studies by Frazie et al, 2000, and Angione et al, 2015.19,20
Given the willingness of biotech and drug delivery device manufacturers to work together to advance safety for patients, Glycome BioPharma has evolved its technology for commercialisation with olefin devices (PFSs, autoinjectors and patch pumps). The one-step covalent surface modification methodology to functionalise olefin polymer surfaces using native human glycans, creates a safe, stable and biocompatible coating that is resistant to leaching/peeling. Critically, this proprietary steric barrier coating has been designed not to compromise device performance or safety.
Comprehensive studies have demonstrated the protein-stabilising properties of this technology and how it can be used to reduce unspecific protein aggregation at the solid-liquid interface. Using quantitative and qualitative fluorescent measurements of treated versus untreated, Glycome BioPharma demonstrated up to 83% and79% reduction in bound insulin and bovine serum albumin respectively on commercially available olefin syringe barrels, a general protein repulsion and functionalisation trend with varying olefin polymer blends (Figure 2). This novel technology commercialisation is timely, given the pressing efforts to the deliver therapeutics to global destinations in the context of our present public health emergency.
REFERENCES
- “FDA Guidance for Industry, Immunogenicity Assessment for Therapeutic Protein Products”. US FDA, Aug 2014.
- Fineberg SE et al, “Immunological responses to exogenous insulin”. Endocr Rev, 2007 Vol 28(6), pp 625–652.
- Creeke PI, Farrell R, “Clinical testing for neutralizing antibodies to interferon-β in multiple sclerosis”. Ther Adv Neurol Disord, 2013, Vol 6(1), pp 3–17.
- Rossert J, Pure Red Cell Aplasia Global Scientific Advisory Board (GSAB), “Erythropoietin-induced, antibody-mediated pure red cell aplasia”. Eur J Clin Invest, 2005, 35 (Suppl 3), pp 95–99.
- Norde W, Lyklema J, “Interfacial behaviour of proteins, with special reference to immunoglobulins. A physicochemical study”. Adv Colloid Interface Sci, 2012, Vol 179–182, pp 5–13.
- Biddlecombe JG et al “Determining antibody stability: creation of solid-liquid interfacial effects within a high shear environment”. Biotechnol Prog, 2007, Vol 23(5), pp 1218–1222.
- Biddlecombe JG et al, “Factors influencing antibody stability at solid-liquid interfaces in a high shear environment”. Biotechnol Prog, 2009, Vol 25 (5), pp1499–1507.
- Waxman L, Vilivalam VD, “A Comparison of Protein Stability in Prefillable Syringes Made of Glass and Plastic”. PDA J Pharm Sci Technol, 2017, Vol 71(6), pp 462–477.
- Perevozchikova T et al, “Protein adsorption, desorption, and aggregation mediated by solid-liquid interfaces”. J Pharm Sci, 2015, Vol 104(6), pp 1946–1959.
- Maruno T et al, “Sweeping of Adsorbed Therapeutic Protein on Prefillable Syringes Promotes Micron Aggregate Generation”. J Pharm Sci, 2018, Vol 107(6), pp 1521–1529.
- Kapp SJ et al, “Competitive adsorption of monoclonal antibodies and nonionic surfactants at solid hydrophobic surfaces”. J Pharm Sci, 2015, Vol 104(2), pp 593–601.
- Maggio ET, “Alkylsaccharides: circumventing oxidative damage to biotherapeutics caused by polyoxyethylene-based surfactants”. Ther Deliv, 2013, Vol 4(5), pp 567–572.
- Huby RD, Dearman RJ, Kimber I, “Why are some proteins allergens?”. Toxicol Sci, 2000, Vol 55(2), pp 235–246.
- Price KS, Hamilton RG, “Anaphylactoid reactions in two patients after omalizumab administration after successful long-term therapy”. Allergy Asthma Proc, 2007, Vol 28(3), pp313–319.
- Limaye S et al, “An allergic reaction to erythropoietin secondary to polysorbate hypersensitivity.” J Allergy Clin Immunol, 200, Vol 110(3), p 530.
- Bonamichi-Santos R, Castells M, “Diagnoses and Management of Drug Hypersensitivity and Anaphylaxis in Cancer and Chronic Inflammatory Diseases: Reactions to Taxanes and Monoclonal Antibodies”. Clin Rev Allergy Immunol, 2018, Vol 54(3), pp 375–385.
- Gadgil HS et al, “The LC/MS analysis of glycation of IgG molecules in sucrose containing formulations”. J Pharm Sci, 2007, Vol 96(10), pp 2607–2621.
- Marchant RE, Yuan S, Szakalas-Gratzl G, “Interactions of plasma proteins with a novel polysaccharide surfactant physisorbed to polyethylene”. J Biomater Sci Polym Ed, 1994, Vol 6(6), pp 549–564.
- Frazie RA et al, “Characterization of protein-resistant dextran monolayers”. Biomaterials, 2000, Vol 21(9), pp 957–966.
- Angione MD et al, “Enhanced Antifouling Properties of Carbohydrate Coated Poly(ether sulfone) Membranes”. ACS Appl Mater Interfaces, 2015, Vol 7(31), pp 17238–17246.
Previous article
ROOM FOR INNOVATION?Next article
I-PLATFORM DEVICE: THE SMART DEVICE WITH APTITUDE