To Issue 159
Citation: Noakes T, Johnson S, Greenhough R, “Navigating the Transition to Sustainable Medical Propellants”. ONdrugDelivery, Issue 159 (Apr/May 2024), pp 44–50.
Timothy Noakes, Sheryl Johnson and Richard Greenhough, discuss the status of the current move from high-global-warming-potential propellants for pressurised metered dose inhalers to more sustainable alternatives.
INTRODUCTION
The pressurised metered dose inhaler (pMDI) has been a major respiratory delivery method since its introduction by Riker Laboratories in 1956 as a new dosage form for adrenaline (epinephrine) and isoproterenol (Figure 1).1 Since then, it has evolved to become arguably the most generic platform technology in the respiratory device space, capable of handling virtually all small molecule respiratory medications.
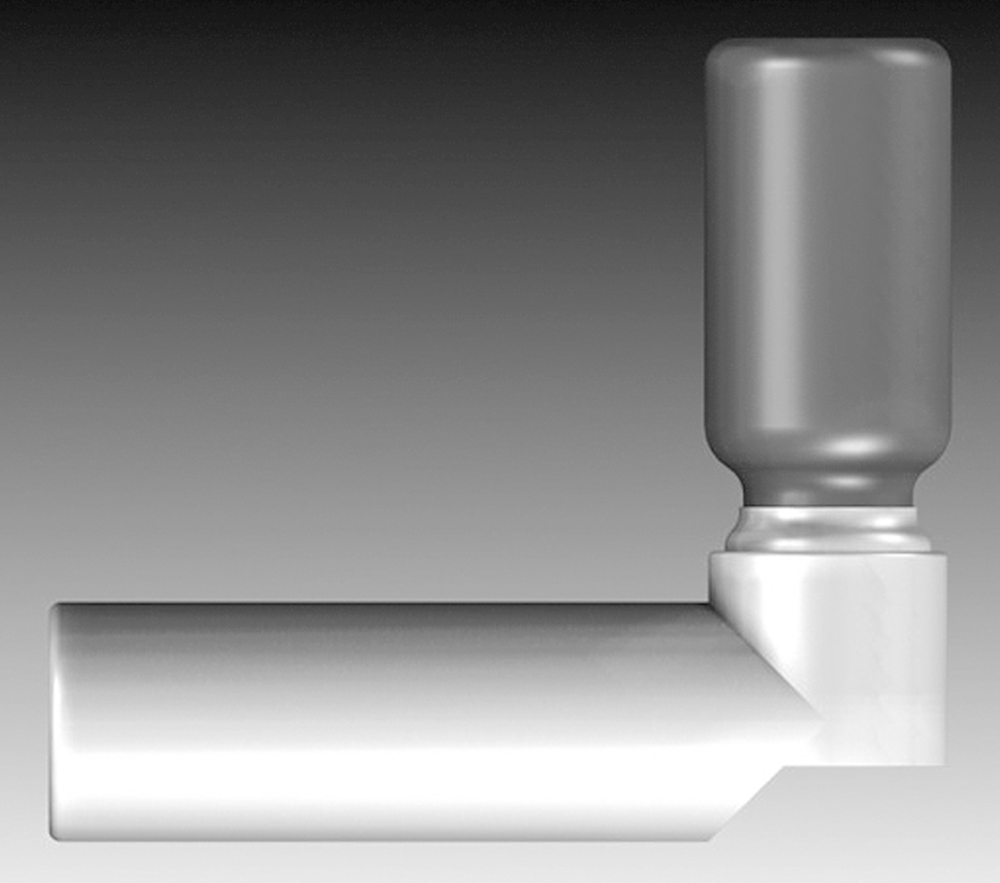
Figure 1: Riker Laboratories’ Medihaler (1956). (Image credit: Mobile Medical Museum and Kindeva.)
To function and deliver a respirable mist, the technology relies on a number of critical components: the metering valve, the actuator and the propellant, (which acts as the drug carrier and can comprise up to 99.9% of the inhaled dose). A successful pMDI propellant must possess several attributes in addition to a low boiling point (typically -30°C to -15°C), it must be relatively dense, inert (especially in people) and have suitable solvent power. This is not an easy combination of properties to achieve, which is why there is near-universal use of partially fluorinated molecules to fulfil this duty.
Between 1956 and 1995, this was achieved with the use of chlorofluorocarbons (CFCs), after which a transition to the current hydrofluoroalkane (HFA) propellants, HFA-134a and HFA-227ea, was initiated. Now the industry is looking at a further change to HFA-152a and HFO-1234ze(E), as summarised in Table 1. Both changes were driven by the introduction of regulations controlling emissions to the environment, addressing ozone depletion and, more recently, combatting global warming.
Propellant | Formula | Boiling point (°C) |
Density (g/mL) |
Ozone depletion potential | GWP (100 years) |
Atmospheric lifetime |
CFC-11 | CCl3F | 23.7 | 1.49 | 1 | 4,750 (AR4) | 45 years2 |
CFC-12 | CCl2F2 | -29.8 | 1.33 | 1 | 10,900 (AR4) | 100 years2 |
HFA-134a | CF3-CFH2 | -26.2 | 1.23 | 0* | 1,430 (AR4) | 14 years2 |
HFA-227ea | CF3-CFH-CF3 | -16.5 | 1.41 | 0 | 3,220 (AR4) | 34 years2 |
HFA-152a | CF2H -CH3 | -24.7 | 0.91 | 0 | 124 (AR4) | 1.4 years2 |
HFO-1234ze(E) | CF3-CH=CHF | -18.9 | 1.29 | 0 | 1.37 (AR6) | 18 days3 |
Table 1: Summary of environmental and physical properties of previous and current medical propellants. *To enable the Kigali amendment to the existing Montreal Protocol regulation to encompass HFA-134a, it has been assigned a small, non-zero ozone depletion potential.
“Unlike CO2 emissions, which are an inherent cost of the combustion of fossil fuels, there is the potential for drop-in replacements of high-GWP fluorocarbons with low-GWP alternatives – a relatively easy solution that is not available to controlling emissions of CO2.”
Although driven by the need to reduce atmospheric ozone depletion (the original Montreal Protocol phased out ozone depleting molecules such as CFCs),4 the introduction of the HFA propellants also resulted in a nearly tenfold reduction in the global warming potential (GWP) associated with pMDI usage. This is due to HFA-134a and HFA-227ea possessing a significantly lower GWP than CFCs (1,430 and 3,220 versus 10,900 for CFC-12 GWP 100-year).5
However, the GWP of these two HFAs is still considerable, and their use has come under regulatory scrutiny in turn. The Kigali Amendment to the Montreal Protocol (2016) introduced measures to reduce the global warming impact of fluorocarbon gases.6 This is because a key component of the effort to slow climate change involves reductions in emissions of special-use greenhouse gases, which, though emitted to a far lesser extent than CO2, are also much more potent in terms of radiative forcing.
Reducing emissions of high-GWP fluorocarbon gases used as propellants is a critical part of this strategy. Unlike CO2 emissions, which are an inherent cost of the combustion of fossil fuels, there is the potential for drop-in replacements of high-GWP fluorocarbons with low-GWP alternatives – a relatively easy solution that is not available to controlling emissions of CO2.
Consequently, several regulatory bodies around the world have been restricting the use of high-GWP fluorocarbons. These efforts include the F-gas regulation in the EU,7 regulations adopted by certain US States8 and US federal requirements9 on the introduction of new alternatives in such applications.
While regulation that addresses man-made global warming contributions has gained most attention, another set of regulatory requirements relating to the escape of classes of fluorocarbons known as per- and poly-fluoroalkyl substances (PFASs) into the environment has been in development. These were originally targeted at substances such as polyfluoroalkanes, including perfluorooctyl bromide. The most advanced discussion is in the EU, where the definition of a PFAS has been substantially expanded and now includes some propellant molecules. However, HFA-152a, a potential low-GWP propellant for pMDIs, is not a PFAS or a PFAS precursor, so has a unique and essential role in meeting the EU’s carbon footprint target and other environmental targets. A consultation period on the draft regulation has attracted over 5,000 responses, and a process of review is now underway.10
It is perhaps worth noting that, while 1234ze(E), HFA-134a and HFA-227ea fall within this very broad structural PFAS definition, these fluorocarbon gases are distinctly different chemicals from the longer chain, higher molecular weight chemicals that are currently under increasing regulatory pressure by PFAS regulation. Unlike the PFAS chemicals of concern, gases such as HFA-134a have short biological half-lives in the order of minutes or hours rather than months or years.11 This eliminates the potential for bioaccumulation, particularly in the protein-rich tissues of fish or humans, which is one of the significant concerns about PFASs. This behaviour makes them more analogous to modern fluorinated anaesthetics, such as sevoflurane or desflurane, that are similarly rapidly eliminated from the body.
The new propellants were carefully chosen to comply with the projected trajectory of regulations. Whilst both HFA–152a and HFO–1234ze(E) still contain some fluorine to provide access to those key physical properties, they have a much lower environmental impact, primarily due to their shorter atmospheric lifetimes.
However, before these potential medical propellants could be taken into full development, a critical investigation into whether their toxicology supported their use as inhalation propellants had to be undertaken. Extensive non-clinical toxicology studies, which are now nearing completion, have been underway for several years.12,13
So, attention now moves to bringing low-GWP pMDIs to market. There are questions as to whether, in a broader environmental perspective, they are competitive with alternative technologies, such as dry powder inhalers (DPIs) and soft mist inhalers, whether the increased flammability can be accommodated in a good manufacturing practices (GMP) facility and if metering valves can be easily adapted to suit them.
LIFECYCLE ANALYSIS
While it is tempting to focus purely on the headline GWP figures for the propellants, it is important to take more comprehensive methods into account, incorporating the total environmental impact of a pMDI – particularly when comparing propellants and benchmarking them against propellant-free technologies, such as DPIs. Lifecycle analysis (LCA) is a recognised methodology for assessing the cradle-to-grave environmental impacts associated with a technology. For a pMDI, this should include routes of manufacture for the propellant and device components, patient use and the fate of the inhaler at end-of-life, including the potential for component recycling. The only exception is with respect to API production, as this is considered independent of the delivery platform. The full scope of the lifecycle stages accounted for as part of the LCA methodology is defined in Figure 2.
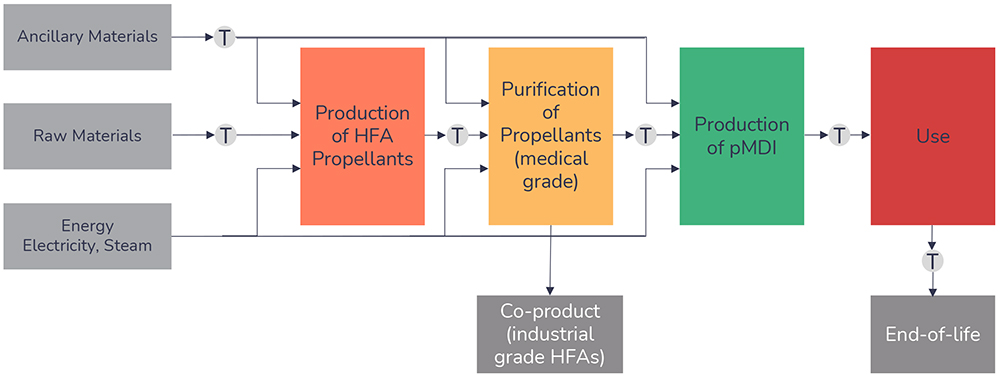
Figure 2: Lifecycle stages of a pMDI.
LCA studies compliant with current environmental management assessment guidelines, such as ISO 14040 and 14044, have been completed for both low-GWP propellants and compared with results for the current medical propellant, HFA-134a. Transition of a pMDI from HFA-134a to HFA-152a has been shown to result in a 90%–92% reduction in environmental impact14 and, when focusing solely on a triple-therapy pMDI (budesonide/glycopyrronium/formoterol fumarate dihydrate), HFA 1234ze(E) resulted in an overall reduction of 85%.15
“Alternatively, transitioning from a current pMDI to a DPI can account for a reduction in around 96%, highlighting that, irrespective of the individual GWP values, a pMDI device containing either will have an environmental impact equivalent to that of a typical DPI.”
Alternatively, transitioning from a current pMDI to a DPI can account for a reduction in around 96%,14 highlighting that, irrespective of the individual GWP values, a pMDI device containing either will have an environmental impact equivalent to that of a typical DPI. To reduce the environmental impact of such inhalers further, be it a DPI or a pMDI, widespread adherence and implementation of device recycling schemes needs to be considered – something that does not exist today.
RECYCLABILITY
The prevalence of inhaler prescriptions for asthma and chronic obstructive pulmonary disorder varies significantly by region. For example, 70% of prescriptions in the UK are for pMDIs, whereas in Sweden this figure is only 13%.16 Nevertheless, all inhalers contain plastics, metals and, in the case of pMDIs, HFAs – with the environmental impact well documented.
In England a small portion of used inhalers are returned to pharmacies. However, it is thought that over 90% are disposed of in household waste.17 Both GSK and Teva have previously funded inhaler recycling schemes,18,19 returning used inhalers to community pharmacy drop-off points. Both schemes had low uptake, however, thought to be due to a lack of public awareness.
Recently, Chiesi trialled “Take Air” in England.20 Remaining propellant from pMDIs was recovered and used in the refrigeration and air conditioning industries, as well as incinerated to provide energy from the waste. Parts were recycled where possible. Around 15,000 pMDIs were returned, which represented 77% of the total inhalers. This saved the equivalent of an estimated 119.3 tonnes of CO2 equivalent emissions into the atmosphere.
The challenge with all schemes, whether by GSK, Teva or Chiesi, is the scalability of such recycling schemes. Further success will rely on scheme publicity, pharmacy and community participation and, undoubtedly, an investment plan put forward by pharmaceutical companies.
“From a pMDI manufacturing perspective, the technology and safety standards for the safe filling, handling and transportation of aerosol products using flammable propellants, including hydrocarbons and HFA-152a, already exist.”
FLAMMABILITY AND MITIGATION
Unlike HFA-134a or HFA-227ea, low-GWP propellants tend to bring the system closer to flammability. In the case of 1234ze(E) it hovers on the border – non-flammable at room temperature, flammable above 30°C. HFA-152a is flammable in air within the concentration range of 3.7%–18.0%. However, computational fluid dynamic modelling has shown that the potential for ignitionof HFA-152a from a pMDI either during use or due to accidental release is negligible.21 This is further confirmed by the fact that many current HFA-134a-based pMDI formulations are actually flammable with no apparent adverse effect on patient safety.
From a pMDI manufacturing perspective, the technology and safety standards for the safe filling, handling and transportation of aerosol products using flammable propellants, including hydrocarbons and HFA-152a, already exist. Indeed, manufacturers of specialist pMDI filling equipment are ready to supply flameproof versions. In addition, a recently released guide is available, authored by three companies in the pMDI industry that have flameproof propellant handling expertise. This guide covers all the key aspects of safe pMDI manufacturing using flammable propellants.22 It must be stressed that this is standard, very well-known and understood aerosol filling technology.
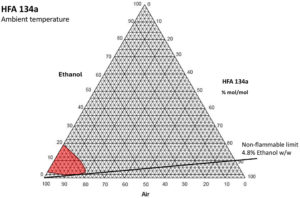
Figure 3: 134a ethanol binary flammability diagram.
Excipients can also change the picture. It is incorrect to assume that if a non-flammable propellant is used, then mixtures containing it and other excipients, both in the manufacturing vessel and in the aerosol, will also be non-flammable. It has been shown that the addition of modest amounts of ethanol to HFA-134a, which is normally non-flammable in any air mixture, will produce a vapour mixture that can be flammable when mixed with air. A flammability diagram demonstrates this (Figure 3).
As can be seen, more than 4.8% ethanol w/w in solution in HFA-134a can produce a flammable vapour cloud when mixed with air at room temperature. This is quite a modest amount, as pMDI formulations that use ethanol typically have 5–15% w/w added. This means that, if such mixtures are created in the filling process, they must be risk assessed, contained and handled as compressed flammable gases.
In light of this, care must be taken when formulation strategies involving ethanol are considered with any medical propellant, in case the mixture flammability boundary is unknowingly crossed, and the formulation becomes flammable, as the manufacturing installation may not have been risk assessed for this eventuality. HFA-152a is well understood and the 4.8% ethanol limit for HFA-134a is known but, at present, there is no relevant data in the public domain regarding HFA-227ea or HFO-1234ze(E). This is a concern when pressure-fill technology is used, as the contents of a pressurised mixing vessel could become flammable if there was some form of escape.
While the cost of building a green-field pMDI filling facility can increase only marginally if it is specified as flameproof from the start, retrofitting existing facilities to become flameproof can be costly. A very useful way of minimising this cost is selecting the right pMDI filling strategy. Traditionally, there are a number of strategies and variants but, for simplicity, these can be categorised into two – two-stage filling (Figure 4) and pressure filling (Figure 5).
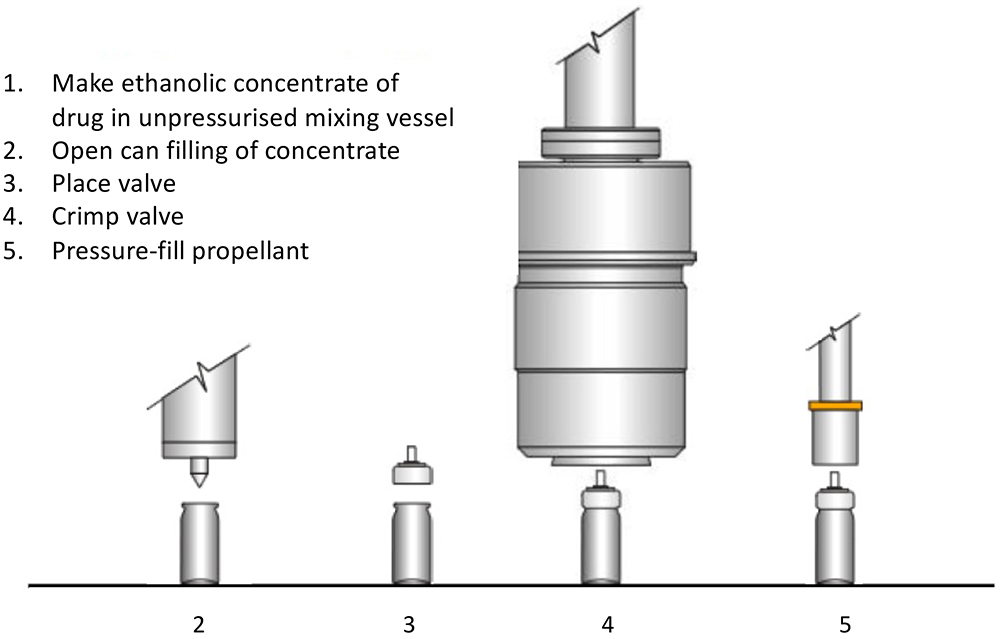
Figure 4: Two-stage pMDI filling process.
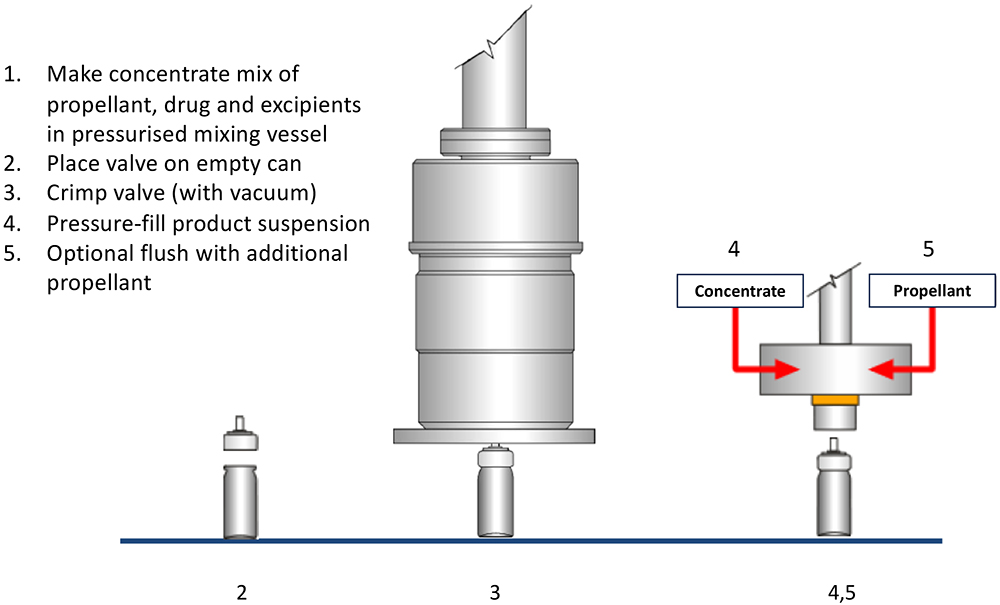
Figure 5: Pressure pMDI filling process.
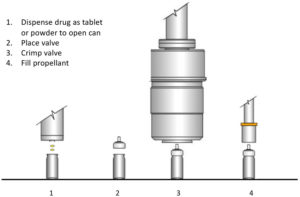
Figure 6: Two-stage tablet/powder pMDI filling process.
The pressure filling option is arguably the most popular with today’s propellants, with two-stage filling being seen as a little limited and unable to fill certain drugs. However, when contemplating a retrofit to handle either flammable propellant or formulations, pressure filling tends to require much more extensive building and equipment alterations than two-stage filling, due to the need for safe accommodation of the pressurised mixing vessel.
Two alternative recent innovations now provide a variant of two-stage filling that works for all required active ingredients via the form of a tablet or powder (Figure 6). The alternate enabling technologies are the use of tablets23 – loose compressed pellets of drug and lactose – or direct dry drug powder dosing into an open canister.24 With the availability of regular ethanol carrier-based two-stage filling plus these alternatives, two-stage filling may become the preferred filling strategy for retrofitting pMDI installations to low-GWP propellants, at a significantly reduced capital cost.
COMPONENTS
“The metering valve, a critical component, requires meticulous fine tuning, which must follow the path of evolution of the formulation.”
Changing the propellant in a pMDI is a challenging process, which needs to take a variety of different technical aspects into consideration. Figure 7 illustrates these aspects, linked to the development, use and commercialisation of a pMDI. The metering valve, a critical component, requires meticulous fine tuning, which must follow the path of evolution of the formulation. This adjustment considers the valve’s sub-components and other crucial elements of the container closure system, as well as the intended manufacturing processes. Aptar Pharma, Bespak and RxPack (Oggiono, Italy) are all major pMDI component manufacturers. These three major companies have a valve offering and all have contributed to investigating how the transition to low-GWP propellants affects performance.
Aptar Pharma, being a drug delivery solutions manufacturer, has played a vital role in the investigation of new materials compatible with the shift towards low-GWP pMDIs. This ensures the availability of devices that work seamlessly with the leading low-GWP propellants – HFA-152a and HFO-1234ze(E). Notably, materials like cyclo-olefin copolymer and ethylene propylene diene monomer rubbers have proven effective as static and dynamic seals in valves, respectively. These rubbers are compatible with both types of propellants, including formulations containing ethanol. In addition, minimising extractables and leachables from components has been a key factor in the selection of new materials.
Adjustments to the metering chamber volume may also be necessary to ensure the delivery of an equivalent dose compared with existing pMDI products. Aptar Pharma has refined its metering chamber design for greater flexibility and robustness, enhancing the support of the dynamic elastomers (Figure 7).
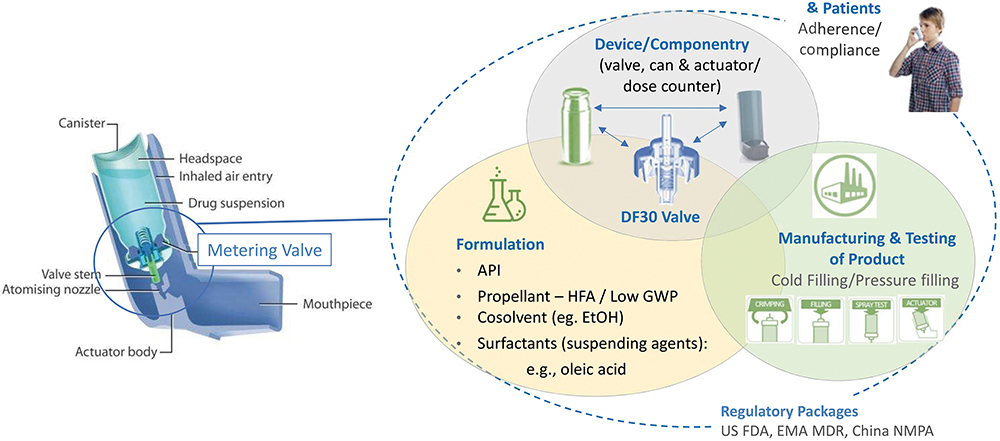
Figure 7: Summary of development strategy for Aptar Pharma valves. (Image credit: Aptar Pharma.)
CONCLUSION
Undoubtedly, the pharmaceutical industry is in the early stages of the transition to low-GWP medical propellants and recognises that there is a possibility that not all existing pMDI products will make the transition successfully, be it for practical or economic reasons. Nevertheless, the transition to a new low-GWP propellant system is happening and could help to invigorate the development of new products or make some products, previously discounted for pMDI use due to environmental factors, a more viable option.
A select number of companies have already announced their intentions to make pMDI products using new low-GWP propellants, which are expected to enter the market from 2025. Given the timelines associated with product development and regulatory approval, as well as further anticipated changes in the environmental regulation, it does seem reasonable that by 2030–2035, a large proportion of the pMDI products in current regulated markets will have completed the transition.
REFERENCES
- Stein SW, Thiel CG, “The History of Therapeutic Aerosols: A Chronological Review”. J Aerosol Med Pulm Drug Deliv, 2017, Vol 30(1), pp 20–41.
- McCulloch A, “Determination of Comparative HCFC and HFC Emission Profiles for the Foam and Refrigeration Sectors until 2015 – PART 3: Total Emissions and Global Atmospheric Concentrations”. US Environmental Protection Agency, 2006.
- “The environmental alternative to traditional refrigerants”. Honeywell, 2012.
- “About Montreal Protocol”. Web Page, UN Environment Programme, Accessed Apr 2024.
- “Global Warming Potential Values”. Greenhouse Gas Protocol, 2016.
- “Introduction to the Kigali Amendment”. OzonAction, 2017.
- “About F-gases”. Web Page, European Commission, Accessed Apr 2024.
- “GHGs Descriptions & Sources in California”. Web Page, California Air Resources Board, Accessed Apr 2024.
- “American Innovation and Manufacturing Act of 2020”. US Code, Title 42, pp 7383–7392.
- “Registry of restriction intentions until outcome”. Web Page, European Chemicals Agency, Accessed Apr 2042.
- Emmen HH et al, “Human safety and pharmacokinetics of the CFC alternative propellants HFC 134a (1,1,1,2-tetrafluoroethane) and HFC 227 (1,1,1,2,3,3, 3-heptafluoropropane) following whole-body exposure”. Regul Toxicol Pharmacol, 2000, Vol 32(1), pp 22–35.
- Mohar I et al, “Overview of the Nonclinical Safety Program for1,1-Difluoroethane (HFA-152a) as a Low Global Warming Potential Metered Dose Inhaler Propellant”. Poster presented at DDL 2023, Dec 2023.
- “SOLSTICE® AIR HFO-1234ze(E), cGMP”. Product pamphlet, Honeywell, 2022.
- Jeswani H, Corr S, Azapagic A, “Reducing carbon footprints of metered dose inhalers”. Inhalation, Dec 2017.
- Hargreaves C et al, “S60 A new medical propellant HFO-1234ze(E): reducing the environmental impact of inhaled medicines”. Thorax, 2022, Vol 77, pp A38–A39.
- Janson C et al, “Carbon footprint impact of the choice of inhalers for asthma and COPD”. Thorax, 2020, Vol 75(1), pp 82–84.
- Sivarajasingam V, “Understanding patients’ knowledge of inhaler recycling”. BJGP Life, Oct 2021.
- “GlaxoSmithKline ‘complete the cycle’ Inhaler Recycling and Recovery Scheme now accessible UK-wide”. Nursing Times, Feb 2013.
- “We’re putting ESG at the heart of everything we do as a business”. Web Page, Teva Ireland, Accessed Apr 2024.
- Murphy A et al, “Understanding the feasibility and environmental effectiveness of a pilot postal inhaler recovery and recycling scheme”. NPJ Prim Care Respir Med, 2023, Vol 33(1), Article 5.
- “Risk of flame from HFA-152a used in metered dose inhalers assessed by CFD modeling and fault tree analysis”. Prepared for Corr S, Mexichem Fluor, Oct 17, 2016 (revised Mar 4, 2020). Copy obtainable from Koura.
- “Zephex 152a – Safe Handling Guide for pMDI Manufacturers (Issue 1)”. Pamasol, Koura, and Recipharm, 2023. Obtainable from https://forms.office.com/e/iZKdZKuhzZ.
- Taylor G, Warren S, Tran C, “Pressurised metered dose inhalers and method of manufacture”. Patent WO2015121653A1, 2015.
- Johnson S et al, “Assessing the adaptability of bench scale dry powder technology for filling of an pMDI can with a high fill weight API”. Poster presented at DDL 2022, Edinburgh, UK, Dec 2022.