Citation: Lee CH, Lee EK, “Skin-Mountable Flexible Needle Patch for Minimally Invasive Controlled Drug Delivery. ONdrugDelivery Magazine, Issue 97 (May 2019), pp 22-25.
Chi Hwan Lee and Eun Kwang Lee introduce a skin-mountable flexible needle patch and discuss its applications in non-invasive drug delivery applications.
INTRODUCTION: MICRO-AND NANO-SCALE NEEDLES
Minimally invasive injection of therapeutics, including biomolecules, into living cells or tissues is a crucial element of various controlled drug delivery systems.1 Research on effective injection methodologies for biomolecules should consider:
- Ensuring minimal risk to cellular survival and function
- Delivery of broad range of biomolecule types with controlled dosage.2
Conventional needle injection penetrates the stratum corneum, the outermost layer of the skin which acts as a barrier for the dermis and epidermis, allowing direct delivery of drug molecules into the vascular circulation.3 This method yields a prompt therapeutic response. However, a challenge remains with the size of commercially available hypodermic needles, typically ranging from millimetre to centimetre, which can be difficult for patients to use themselves, due to pain caused by improper handling or reluctance caused by needle phobia. Especially in developing countries, spread of bloodborne pathogens by needle re-use is also a major issue.4 Hypodermic needles are therefore primarily utilised by healthcare professionals in a clinical setting or at home by well-trained patients who have learned the correct injection method and safe needle disposal.5 Thus, there is an unmet need for the development of an effective and safe drug delivery system comparable in terms of efficiency with conventional needle-injection methodologies but without its disadvantages.
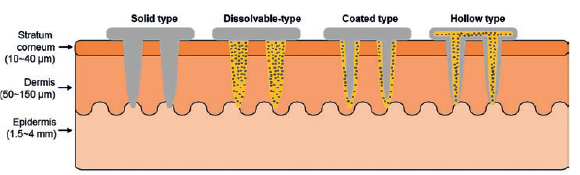
Figure 1: Schematic illustration of various needle forms.
For the minimally invasive injection of biomolecules, recent approaches involve the use of micro- or nano-scale needles capable of penetrating the skin or into cells at their required length scale.6 The incorporation of nanoscale textures on the surface of needles, where drug molecules are bonded, can increase the drug loading capacity, providing high-throughput delivery.7 Examples of different types of micro/nanoneedles are summarised in Figure 1.8
- Solid needles without active pharmaceutical ingredient (API) are used for skin pre-treatment. The solid nanoneedles are applied to the desired skin site and removed, leaving micron-scale holes in the skin. Then ointment or medical creams are applied and cross slowly through the pores.
- Dissolvable needles made of water-soluble or biodegradable polymers that contain drug molecules are used for direct drug delivery. The key benefit of this needle form is that there remain no sharp scars after the needles are completely degraded in the skin.
- Coated needles are solid needles coated with dissolvable polymers that contain drug molecules. The stronger physical properties of this type of needle enable deeper penetration into the skin.
- Hollow needles are manufactured with empty spaces inside them into which liquid drug molecules are filled, ready for delivery into the skin after injection. Hollow nanoneedles can contain high molecular weight compounds such as proteins, vaccines and oligonucleotides.
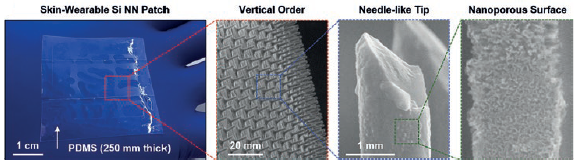
Figure 2: Photograph of a representative Si NN-patch (left) and magnified images of the tips and the nanoporous surface.
More recently, vertical silicon nanoneedles (Si NNs) have been used for intracellular and intratissue delivery of biomolecules, providing further advantages from their intrinsic bio-dissolvability.7 The resultant platform allows the nanoneedles to interact with adjacent cells and tissues during/after the injection, without inducing toxicity, degradation of cell metabolism rupture, followed by complete dissolution in a harmless manner.7 Due their nano-scale size, Si NNs can penetrate the skin without causing pain or damage.
Si NNs are constructed on Si wafers, which provides compatibility with existing Si processing methods and facilities. The sizes, shapes and configurations of Si NNs can be precisely controlled in order to tailor them to specific delivery applications.3,5 Nevertheless, a challenge is posed by the flat, rigid surface of Si wafers, which yield a large mechanical mismatch to the soft, curvilinear and dynamic surface of biological systems, thereby inevitably resulting in suboptimal surface contact. There is therefore a need for a mechanically soft, flexible form of vertical Si NNs, enabling their conformal contact with the surface of cells and tissues and thus efficient intracellular and intratissue drug delivery. Our research group recently developed a novel flexible form of vertical Si NN array on a thin elastomer patch (referred to hereafter as an Si NN-patch), which provides mechanical elasticity, optical transparency and cell and tissue compatibility.7 The flexible platform provides unique capabilities to yield a mechanically elastic interaction between the vertical Si NNs and biological cells and tissues, simultaneously enabling direct, real-time imaging of their interactions due to the patch’s transparency.
Figure 2 shows a series of optical images of the Si NN-patch that incorporates a large array (3×3 cm) of vertically ordered Si NNs on a silicone elastomer, such as polydimethylsiloxane (PDMS). These images demonstrate the mechanical flexibility and optical transparency of the Si NN-patch. The magnified images highlight the needle-like sharp tip of Si NNs with nanoscale pores (8–20 nm diameter) on the surface, formed by using the metal-assisted chemical etching (MACE) method,9 leading to a substantial increase of drug-loading capacity.
FABRICATION OF THE SI NN-PATCH
The fabrication of the Si NN-patch began with a Si wafer to form vertically ordered microscale Si pillars (3 μm diameter) by exploiting standard photolithographic patterning and a deep reactive ion etching (DRIE) processes. A partial passivation of the surface of Si pillars, followed by an isotropic dry etching step, allowed the formation of an undercut in the bottom area. A subsequent anisotropic wet etching step was used to reduce the size of the Si pillars down to nano-scale (<1 μm diameter). A thin layer of PDMS was then deposited on the top area of the Si NNs, where an airgap exists between the PDMS and Si wafer. The entire structure was immersed in an organic solvent solution, such as hexane and dichloromethane, allowing the PDMS to absorb the solvent and swell its volume. The swelling of the PDMS enabled the formation of uniform cracks at the bottom undercut areas where the most significant mechanical strains are concentrated. Subsequent annealing in a convection oven at 70°C for approximately one hour returned the swelled PDMS (now incorporating Si NNs on the surface) to its original volume.
A wide range of needle diameters (80 nm to 3 μm) and heights (8–70 μm) were demonstrated in our studies.
INTRACELLULAR NANO-INJECTION UNDER SIMULTANEOUS REAL-TIME OBSERVATION
The mechanically soft and flexible form of the Si NN-patch provides the ability to form a highly elastic interface between Si NNs and biological cells or tissues. Figure 3A shows an example of the Si NN-patch interfaced with MCF7 (breast cancer cell line) cells. The elasticity of the PDMS substrate allows it to efficiently adapt to the deformation of Si NNs induced by the cell movements, yielding no mechanical distortions or fractures, as are often observed using control Si NNs on a standard rigid Si wafer.
In addition, the Si NN-patch is transparent (the optical transparency is 90%), enabling direct, real-time observation of the interaction between Si NNs and cells or tissues. Figure 3B is a series of differential interference contrast (DIC) microscopy images of MCF7 cells interacting with the Si NNs, all captured from 36 hours of continuous monitoring. The experimental demonstrations of minimally invasive injection and delivery of biomolecules (siRNA) into various kinds of biological cells and tissues provide the feasibility and validity evidence.7
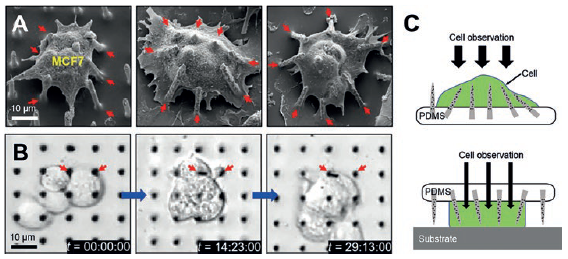
Figure 3: SEM images of tilted Si NNs on a PDMS substrate interacting with a MCF7 cell (A), real-time observation of the MCF7 cells through the transparent PDMS substrate (B) and schematic illustrations of the possible injection schemes from either top or bottom of cells (C).
Figure 3C is a schematic illustration of the possible injection scheme of the Si NNs into cells from either top or bottom, for tailored applications. Due to the transparency of the Si NN-patch, this platform enables systematic studies of the biological interactions between cells and Si NNs observed through the substrate in detail in vivo as well as in vitro.
INTRATISSUE NANO-INJECTION IN AN ANIMAL MODEL
In vivo nano-injection of biomolecules into tissues is also possible using either a skin-wearable or implantable variant of the Si NN-patch. Figure 4 shows sets of photographs and the corresponding in vivo imaging system (IVIS) images in which the Si NN-patch is applied on the skin (4A) and subcutaneous muscle (4B) of mice, respectively. The Si NN-patch provides excellent biocompatibility for integration with the skin and subcutaneous muscle without provoking any inflammation responses. The contact quality at the interface between the patch and the skin or muscle undergoes negligible changes even with motion of the awake mice due to the substantial resilience of the Si NN-patch, which is able to tolerate the mechanical deformation. These aspects enable minimally invasive, uniform injection of small molecules throughout the curved spinal regions.
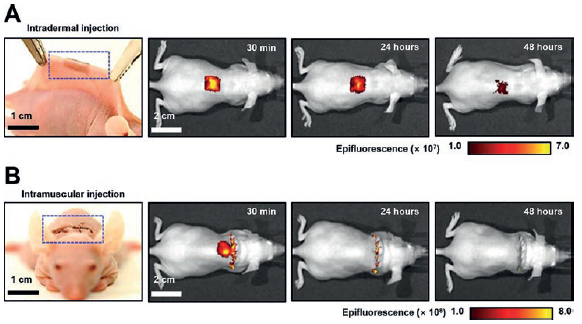
Figure 4: Demonstrations of intradermal (A) and intramuscular (B) injection of the Si NN-patch in mice. The in vivo imaging system (IVIS) images display the diffusion of small-molecule dyes through the skin and muscles after injection.
CLOSING REMARKS
Drug injection using micro- or nano-scale needles in a minimally invasive fashion provides many advantages, such as avoiding significant tissue damage and associated side effects, justifying the recent considerable interest in the field. As detailed in this article, an advanced engineering technique has emerged, enabling the heterogeneous integration of vertically ordered Si NNs containing biomolecules of interest with a flexible, transparent layer of silicone elastomer. The resultant platform provides not only mechanical flexibility to provide conformal contact with the curved surface of cells and tissues, but also optical transparency that enables simultaneous real-time observation during drug delivery. The flexible Si NN-patch would enable many fundamental studies on cellular interactions with Si NNs, including on cellular detection, drug discovery and therapeutic effects of drug/gene delivery.
In order for the Si NN-patch to be commercially available, fundamental studies and technological elaboration should proceed together. Research on the synergistic effects of various biomolecules using nanoporous Si NNs has yet to mature, and progress towards fabrication of the Si NN-patch in an industrially manufacturable and cost-effective manner is still in its infancy.
REFERENCES
- Li Y, Rodrigues J, Tomás H, “Injectable and biodegradable hydrogels: gelation, biodegradation and biomedical applications”. Chem Soc Rev, 2012, Vol 41(6), pp 2193–2221.
- Shalek AK et al, “Vertical silicon nanowires as a universal platform for delivering biomolecules into living cells”. Proc Natl Acad Sci USA, 2010, Vol 107(5), pp 1870–1875.
- Lee H et al, “Device-assisted transdermal drug delivery”. Adv Drug Deliv Rev, 2018, Vol 127, pp 35–45.
- Lee JW, Park JH, Prausnitz MR, “Dissolving microneedles for transdermal drug delivery”. Biomaterials 2008, Vol 29(13), pp 2113–2124.
- Kim YC, Park JH, Prausnitz MR, “Microneedles for drug and vaccine delivery”. Adv Drug Deliv Rev, 2012, Vol 64(14), pp 1547–1568.
- Waghule T et al, “Microneedles: A smart approach and increasing potential for transdermal drug delivery system”. Biomed Pharmacother, 2019, Vol 109, pp 1249–1258.
- Kim H et al, “Flexible elastomer patch with vertical silicon nanoneedles for intracellular and intratissue nanoinjection of biomolecules”. Sci Adv, 2018, Vol 4(11), epub.
- Lim DJ et al, “Microneedles: A versatile strategy for transdermal delivery of biological molecules”. Int J Biol Macromol, 2018, Vol 110, pp 30–38.
- Han H, Huang Z, Lee W, “Metal-assisted chemical etching of silicon and nanotechnology applications”. Nano Today, 2014, Vol 9(3), pp 271–304.